References
Published online by Cambridge University Press: 05 June 2014
Summary
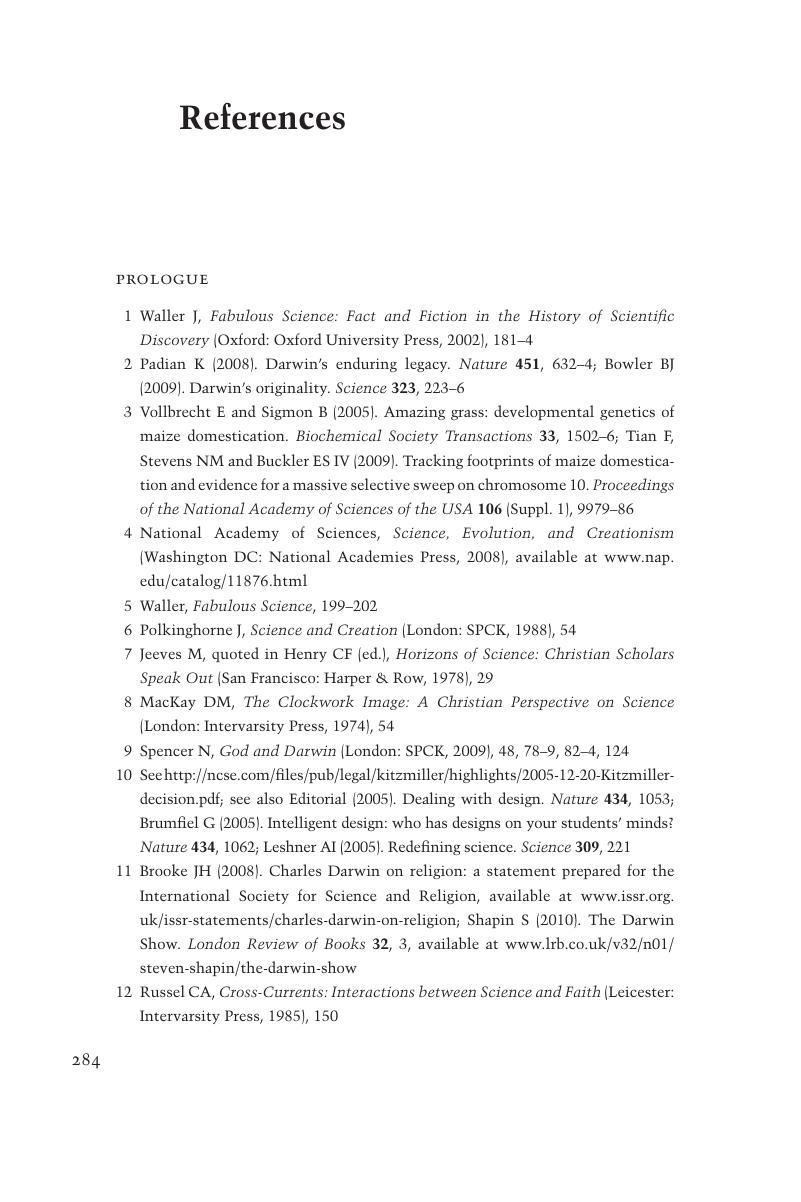
- Type
- Chapter
- Information
- Human EvolutionGenes, Genealogies and Phylogenies, pp. 284 - 350Publisher: Cambridge University PressPrint publication year: 2013
12th August 2024: digital purchasing is currently unavailable on Cambridge Core. Due to recent technical disruption affecting our publishing operation, we are experiencing some delays to publication. We are working hard to restore services as soon as possible and apologise for the inconvenience. For further updates please visit our website: https://www.cambridge.org/news-and-insights/technical-incident
Published online by Cambridge University Press: 05 June 2014
To save this book to your Kindle, first ensure coreplatform@cambridge.org is added to your Approved Personal Document E-mail List under your Personal Document Settings on the Manage Your Content and Devices page of your Amazon account. Then enter the ‘name’ part of your Kindle email address below. Find out more about saving to your Kindle.
Note you can select to save to either the @free.kindle.com or @kindle.com variations. ‘@free.kindle.com’ emails are free but can only be saved to your device when it is connected to wi-fi. ‘@kindle.com’ emails can be delivered even when you are not connected to wi-fi, but note that service fees apply.
Find out more about the Kindle Personal Document Service.
To save content items to your account, please confirm that you agree to abide by our usage policies. If this is the first time you use this feature, you will be asked to authorise Cambridge Core to connect with your account. Find out more about saving content to Dropbox.
To save content items to your account, please confirm that you agree to abide by our usage policies. If this is the first time you use this feature, you will be asked to authorise Cambridge Core to connect with your account. Find out more about saving content to Google Drive.