Introduction
Perinatal stroke causes hemiparetic cerebral palsy (HCP) and lifelong neurological disability. With morbidity spanning diverse aspects of a child’s life and lasting for decades, global impact is large, including 10,000 Canadian children. The burden of disabilities will persist as pathophysiology is poorly understood and prevention strategies are non-existent. Limited treatments lead to loss of hope for children and families, necessitating exploration of new therapies.
As a focal injury in a healthy brain at the start of life, perinatal stroke represents an ideal model for the study of human developmental plasticity. Fortunately, combinations of preclinical and human studies are informing models of how the brain organizes specific functions after perinatal stroke. These models in turn are creating specific targets for neuromodulation where clinical trials are now underway. Advanced neuroimaging has been essential to this entire process, constructing the models to define individual patterns of developmental plasticity and then exploring the effects and potential mechanisms of intervention.
Here, we summarize the approaches, evidence, and emerging directions of how neuroimaging can inform our understanding of both developmental and interventional plasticity, paving the way for neuromodulation trials to improve outcomes for children with perinatal stroke.
Perinatal Stroke: Disease States and Outcomes
You will not incur a higher period of risk for ischemic stroke than the week you are born. Reference Mineyko and Kirton1 A term newborn carries a risk >1:1500, threefold higher than a week in the life of a diabetic, hypertensive, smoking adult and eightfold above all adults. Reference Petty, Brown and Whisnant2 An additional 50% of perinatal stroke presents later in infancy. Reference Raju, Nelson, Ferriero and Lynch3 Perinatal stroke is the leading cause of HCP and most survivors experience additional neurological comorbidities including intellectual disabilities, language impairments, developmental and behavioral disorders, and epilepsy. Reference Sreenan, Bhargava and Robertson4,Reference Kirton5 Frequent occurrence combined with lifelong morbidity generates a large global burden on affected children and their caregivers. Identification of a causative factor remains elusive in most cases Reference Mineyko and Kirton1 and with no means of prevention, perinatal stroke will continue to impact the >10,000 affected Canadian children and their families for decades to come (Dunbar & Kirton, under review).
An essential first step in improving outcomes from perinatal stroke is to understand the underlying disease. We have helped define distinct clinical-radiographic perinatal stroke syndromes using magnetic resonance imaging (MRI), Reference Kirton and deVeber6,Reference Kirton, deVeber, Pontigon, MacGregor and Shroff7 refining perinatal stroke research toward specific disease states. Two main stroke types predominate (Figure 1). Arterial ischemic strokes (AISs) are large brain injuries secondary to occlusion of major cerebral arteries. Some present at birth with acute seizures (symptomatic neonatal AIS), while others are not recognized until infancy when hemiparesis becomes evident (arterial presumed perinatal ischemic stroke). Reference Kirton, deVeber, Pontigon, MacGregor and Shroff7–Reference Kirton, Armstrong-Wells and Chang9 In contrast, periventricular venous infarctions (PVIs) are subcortical white matter lesions acquired in the fetus before 34 weeks gestation, secondary to medullary venous infarction. Reference Kirton, deVeber, Pontigon, MacGregor and Shroff7,Reference Elchalal, Yagel and Gomori10,Reference Takanashi, Barkovich, Ferriero, Suzuki and Kohno11 MRI studies by our group and others have defined these fetal strokes, facilitating diagnosis. Reference Kirton and Wei12,Reference Takanashi, Tada, Barkovich and Kohno13 We have validated this imaging-based classification system and demonstrated its research applications including MRI prediction of long-term outcomes, recognition of novel risk factors, MRI markers of pathophysiology, and new imaging targets for therapeutic interventions. Reference Kirton, deVeber, Pontigon, MacGregor and Shroff7,Reference Kirton, Armstrong-Wells and Chang9,Reference Domi, deVeber and Shroff14–Reference Tuor, Morgunov and Sule18
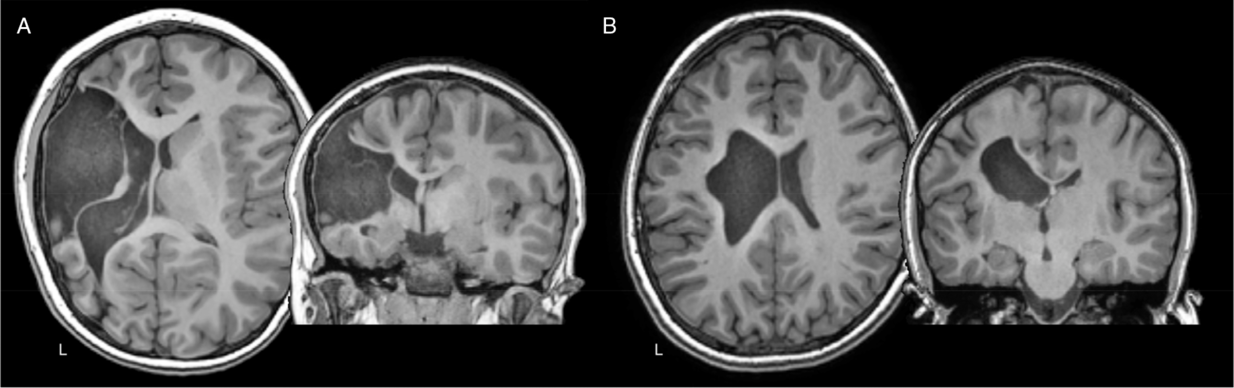
Figure 1: Axial and coronal T1-weighted anatomical images of two teenage children with left hemisphere perinatal strokes. (A) Arterial presumed perinatal ischemic stroke (APPIS). (B) Periventricular venous infarction (PVI). Both children have significant motor disabilities (hemiparetic cerebral palsy) that will last a lifetime.
Both AIS and PVI injure primary components of the motor system early in life, often resulting in HCP. Neurodevelopmental deficits occur in ~75% of perinatal stroke survivors. Reference Sreenan, Bhargava and Robertson4,Reference Kirton5,Reference Mercuri, Barnett and Rutherford19 Motor deficits are most prominent and are not limited to one body side. Reference Kirton5,Reference Kirton, deVeber, Pontigon, MacGregor and Shroff7,Reference Lee, Croen and Lindan20–Reference Trauner, Chase, Walker and Wulfeck22 Ability to predict motor outcome early is limited, but MRI has dramatically improved the early identification of affected children, Reference Kirton, deVeber, Pontigon, MacGregor and Shroff7,Reference Mercuri, Barnett and Rutherford19,Reference Lee, Croen and Lindan20,Reference Boardman, Ganesan and Rutherford23,Reference Mercuri, Rutherford and Cowan24 including our use of diffusion MRI to predict hemiparesis. Reference Kirton, Domi, Shroff, Kouzmitcheva and deVeber15,Reference De Vries, Van der Grond, Van Haastert and Groenendaal25,Reference van der Aa, Verhage and Groenendaal26 This has opened windows for earlier intervention. Deficits in language, vision, cognition, behavior, and epilepsy complicate >50% of AIS with morbidities lasting a lifetime. Physical disability remains the main determinant of quality of life, and current interventions are limited. Reference Bemister, Brooks, Dyck and Kirton27,Reference Friefeld, Westmacott, MacGregor and deVeber28
Plastic Organization Following Perinatal Brain Injury
The common features of unilateral, focal injury in an otherwise healthy brain, combined with distinct differences in lesion timing and location, make perinatal stroke an ideal human model for the study of developmental plasticity. Reference Kirton29 In 1936, Kennard described better outcomes in younger primates following motor cortex lesions. Reference Kennard30 This Kennard principle has fostered efforts to understand and harness age-related plasticity. Terms like “repair” and “reorganization” imply the existence of inherent restorative mechanisms that evolutionary models suggest do not exist. Reference Trevathan, Trevathan, Smith and McKenna31 Instead, plastic adaptation represents ongoing developmental and compensatory processes occurring after injury.
Elegant animal work and human studies have solidified a model that increasingly explains motor function following early unilateral injury, creating novel avenues for therapeutic interventions. Reference Kirton29,Reference Eyre32–Reference Staudt34 These will be outlined here to then demonstrate how advanced imaging has both informed progress and possesses new potential to expand our understanding.
Synaptic Competition
The targets of developing upper motor neuron systems are the spinal lower motor neurons, control of which appears to be a major determinant of clinical function. In typical development, ipsilateral and contralateral corticospinal tract (CST) projections arising from primary motor cortex (M1) are present in equal proportion at birth (Figure 2A). With continuous competition to establish synapses occurring through development, eventual contralateral domination results and ipsilateral projections have been shown to physically withdraw in animal models (Figure 2B). Reference Eyre32,Reference Eyre, Taylor, Villagra, Smith and Miller35,Reference Muller, Kass-Iliyya and Reitz36 In humans, these connections are also thought to largely withdraw during the first 2 years of life, leaving few (~15%), slower conducting ipsilateral projections. Reference Eyre32 Evidence from transcranial magnetic stimulation (TMS) studies in neurologically typical children (aged 8–18 years) are consistent and have found few detectible motor evoked responses in the ipsilateral hand. Reference Zewdie, Damji, Ciechanski, Seeger and Kirton37 Continued motor practice during development strengthens contralateral connections, with plastic changes in M1 occurring in a use-dependent fashion. Reference Salimi and Martin38,Reference Martin and Lee39
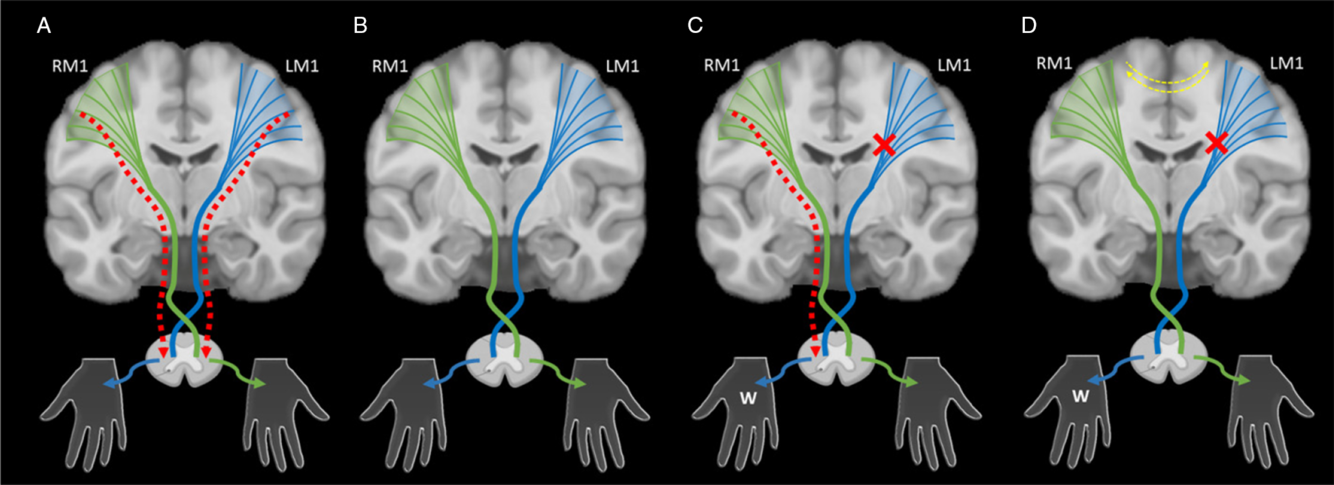
Figure 2: An illustration of the synaptic competition model. (A) In typical development, ipsilateral (red dashed lines) and contralateral corticospinal tract (CST) projections (green and blue lines) arising from primary motor cortex (M1) and broader cortical areas are present in equal proportion at birth. They compete with each other to establish synapses with lower motor neurons during early development. (B) With normal motor development, contralateral projections dominate and ipsilateral projections are withdrawn. (C) After an early unilateral injury such as perinatal stroke (red x), abnormal ipsilateral projections that would have been withdrawn may persist (red dashed line), resulting in ipsilateral (or bilateral) control of the weak hand (W), a pattern often associated with worse motor function. (D) Models suggest that intervention strategies focused on restoring typical contralateral organization of the motor system might maximize function. Adjuvant neuromodulation techniques may additionally influence this balance at multiple levels including the interhemispheric balance between primary motor cortices (yellow dashed lines). LM1 – left motor cortex, RM1 – right motor cortex.
In the case of perinatal stroke, the injury reduces activity of sensorimotor neurons in the lesioned hemisphere resulting in a failure to maintain activity-dependent contralateral projections subsequently sparing ipsilateral projections. Reference Martin and Lee39 Resulting weakened contralateral competition from the lesioned hemisphere may cause ipsilateral projections that normally withdraw to persist resulting in ipsilateral (or bilateral) control of the weak hand and poorer motor function (Figure 2C).
The Lesioned Hemisphere: Contralateral Projections to the Paretic Hand
Adult stroke and animal studies suggest that retaining motor control in the lesioned hemisphere is associated with better physical function. Reference Ward40,Reference Werhahn, Conforto, Kadom, Hallett and Cohen41 This organization may be most efficacious because if relatively intact perilesional tissue mediates motor function in the paretic hand, existing (albeit damaged) contralateral CST projections are utilized which may be better suited to motor execution compared to weaker or “unintended” ipsilateral pathways. Reference Staudt, Grodd and Gerloff42 We and others have correlated preserved contralateral projections with function. Reference Staudt34,Reference Zewdie, Damji, Ciechanski, Seeger and Kirton37 HCP studies show decreased excitability in the lesioned hemisphere with location of cortical activations approximating typical motor areas. Reference Berweck, Walther and Brodbeck43,Reference Staudt, Gerloff and Grodd44 Contralateral CST orientations also have better function in PVI. Reference Staudt, Grodd and Gerloff42 Small imaging studies suggest rehabilitation may increase lesioned motor cortex activations in HCP. Reference Juenger, Linder-Lucht and Walther45,Reference Walther, Juenger and Kuhnke46 Enhancing motor control in the lesioned hemisphere may favor improved function but markers remain unestablished in perinatal stroke.
The Contralesional Hemisphere: Ipsilateral Projections to the Paretic Hand
Abnormal ipsilateral projections from the non-lesioned hemisphere to the paretic hand are common in HCP (Figure 2C). Reference Carr, Harrison, Evans and Stephens47–Reference Nezu, Kimura, Takeshita and Tanaka50 We and others have confirmed the association between this ipsilateral arrangement and poor function in perinatal stroke Reference Staudt, Grodd and Gerloff42,Reference Carr, Harrison, Evans and Stephens47–Reference Thickbroom, Byrnes, Archer, Nagarajan and Mastaglia51 . Importantly, over-activity of the contralesional M1 may be associated with larger deficits. Reference Staudt, Grodd and Gerloff42,Reference Nezu, Kimura, Takeshita and Tanaka50,Reference Ragazzoni, Cincotta, Borgheresi, Zaccara and Ziemann52 In the largest TMS study of perinatal stroke (n = 52), we validated this neurophysiology of the contralesional M1. Reference Zewdie, Damji, Ciechanski, Seeger and Kirton37
Interhemispheric Dynamics
Interhemispheric inhibition (IHI), whereby each hemisphere inhibits the other, may help to maintain excitatory and inhibitory balance in the motor system. Reference Ferbert, Priori and Rothwell53 IHI between the primary motor cortexes in school-aged children appears to be similar to that observed in adults. Reference Ciechanski, Zewdie and Kirton54 In perinatal stroke, we have shown that less IHI from the stroke to non-stroke M1 is associated with better function. Reference Eng, Zewdie, Ciechanski, Damji and Kirton55 Decreasing over-activity of contralesional M1 has been a commonly used approach to therapeutic neuromodulation in adult stroke. Reference Hsu, Cheng, Liao, Lee and Lin56,Reference Kang, Summers and Cauraugh57 Such techniques may modulate cortical excitability to restore interhemispheric balance between the primary motor cortices (Figure 2D, yellow dashed lines) and thus represent a potential therapeutic target in HCP.
Modulating Developmental Plasticity in Cerebral Palsy
Interventional strategies may enhance contralateral (or inhibit ipsilateral) CST projections to improve physical function. There is strong animal evidence to support activity-dependent enhancement of contralateral CST connections. Reference Salimi and Martin38,Reference Grefkes and Fink58 These findings complement evidence in human histopathological and neurophysiological studies including demonstration in healthy and injured newborns and infants. Reference Eyre, Taylor, Villagra, Smith and Miller35,Reference Martin, Kably and Hacking59,Reference Scales and Collins60 Leading interventional approaches for children with HCP build on evidence from adult stroke, namely manual therapies and non-invasive brain stimulation of cortical areas.
Manual Therapy
Manual therapies are designed to deliver motor learning-based therapy, the essential substrate for use-dependent plasticity. Limited use of the contralesional limb reduces opportunity for strengthening desirable contralateral CST connections, calling for therapies that focus on actively engaging children in intensive, repetitive, functional motor practice. The bulk of research to date has focused on constraint-induced movement therapy (CIMT). CIMT promotes use of an impaired limb by constraint of the less-impaired limb, Reference Taub, Crago and Uswatte61 with multiple pediatric studies supporting CIMT effectiveness in HCP. Reference Eliasson, Krumlinde-Sundholm, Shaw and Wang62–Reference Novak, Morgan and Fahey65 In adult stroke, 2 weeks of CIMT can generate gains lasting years. Reference Wolf, Winstein and Miller66 Limitations of CIMT include a somewhat invasive nature and the exclusion of bimanual learning, which is arguably more useful for children’s everyday life and may target sensorimotor deficits observed in function of both hands. Reference Kuczynski, Carlson and Lebel67,Reference Rich, Menk, Rudser, Feyma and Gillick68 Bimanual approaches provide interventional options that can also improve function in HCP, Reference Novak, Morgan and Fahey65,Reference Gordon, Schneider, Chinnan and Charles69 with comparable benefits to CIMT. Many programs now combine these methods. Reference Gordon, Hung and Brandao70 Response patterns vary with continued efforts to identify individual factors that may guide intervention selection. Reference Schertz, Shiran and Myers71
Non-Invasive Brain Stimulation
Transcranial Magnetic Stimulation
TMS uses electromagnetic induction to apply a suprathreshold pulse to focal cortical areas. TMS has high spatial resolution to affect discrete functional areas, offering non-invasive, painless mapping of motor systems. Reference Carr, Harrison, Evans and Stephens47,Reference Garvey and Gilbert72–Reference Rajapakse and Kirton74 TMS neurophysiology procedures can quantify motor pathway integrity and cortical excitability Reference Zewdie, Kirton, Kirton and Gilbert75 and can elucidate interactions between different brain areas. Advances with TMS robotic measurements Reference Ginhoux, Renaud and Zorn76 offer methods to assess dynamic changes in M1 that may occur following interventions. Reference Friel, Kuo and Fuller77 That non-invasive brain stimulation that can produce lasting changes in brain function is now well established. Reference Lefaucheur, André-Obadia and Antal78 TMS is amenable to randomized, sham-controlled clinical trials with dozens in adult stroke demonstrating improved function. High-frequency repetitive pulse TMS (rTMS) stimulates cortex which both animal Reference Adkins-Muir and Jones79–Reference Teskey, Flynn, Goertzen, Monfils and Young81 and human Reference Hsu, Cheng, Liao, Lee and Lin56 stroke studies can facilitate motor function. Low-frequency rTMS often reduces the excitability of the cerebral cortex Reference Chen, Classen and Gerloff82,Reference Pascual-Leone, Amedi, Fregni and Merabet83 and contralesional application may enhance stroke recovery in adults Reference Hsu, Cheng, Liao, Lee and Lin56 and hand function in HCP, Reference Kirton, Chen and Friefeld84,Reference Gillick, Krach and Feyma85 with additive effects of CIMT and rTMS. Reference Kirton, Andersen and Herrero86 Strong evidence supports rTMS safety in both adult and pediatric stroke trials including no adverse effects on hand function. Reference Hsu, Cheng, Liao, Lee and Lin56,Reference Gillick, Krach and Feyma85–Reference Zewdie, Ciechanski and Cherie Kuo89 Limitations of rTMS include burdensome, immobile hardware that prevents simultaneous rehabilitation.
Transcranial Direct Current Stimulation
Transcranial direct current stimulation (tDCS) applies simple scalp electrodes (anode and cathode) to generate weak currents (1–2 mA) that induce polarity-dependent changes Reference Zaghi, Acar, Hultgren, Boggio and Fregni90 and regional modulation of resting membrane potential and neuronal excitability. Reference Nitsche and Paulus91 Anodal stimulation generally increases excitability, while cathodal stimulation decreases it but there are exceptions. Reference Dayan, Censor, Buch, Sandrini and Cohen92 tDCS safety and tolerability in adults Reference Bikson, Grossman and Thomas93 and children Reference Zewdie, Ciechanski and Cherie Kuo89 are well established, and the portable nature of tDCS systems allows patient mobility during active rehabilitation, providing opportunity to potentially augment motor practice with concurrent neurostimulation. Motor cortex tDCS can enhance motor learning in animals, healthy adults and children, and adults with stroke during training. Reference Fritsch, Reis and Martinowich94–Reference Ciechanski and Kirton97 Investigations in children with HCP suggest benefit of combining tDCS with motor training. Reference Kirton, Ciechanski and Zewdie98,Reference Gillick, Rich and Nemanich99 Use of tDCS and TMS seem promising, but mechanisms of action are not yet well understood.
Across intervention approaches, missing is an understanding of the underlying plastic changes that drive improvements in hand function following manual therapies and neuromodulation. This is required to identify individual factors that predict responsiveness to generate personalized neurorehabilitation to optimize function.
Neuroimaging
MRI affords incredible opportunities to explore how brain structure and function change as children with perinatal stroke grow (developmental plasticity) and to better understand the effects of training and neuromodulation (interventional plasticity). Application of advanced MRI techniques in perinatal stroke will allow us to further understand the brain’s structure, function, and metabolism to identify individual markers with which to design targeted, personalized interventions. With the exciting advent of large publicly available imaging databases (including pediatric databases), future developments may establish large, normative comparison groups to further development of personalized neuroimaging biomarkers that can inform rehabilitation strategies in individual patients.
Structural Imaging
Perhaps the simplest method of imaging perinatal stroke is structural MRI. A standard T1- or T2-weighted anatomical image can provide a myriad of information regarding lesion location, character, and size as well as structural volumes, tissue signal intensity, thickness of cortical areas, and cortical complexity (i.e., gyrification or sulcal depth). Clinically obtained anatomical scans, including T2-weighted fluid-attenuated inversion recovery, often help classify the majority of stroke subtypes based on infarct shape, territory, and associated features. Reference Kirton, Deveber, Pontigon, Macgregor and Shroff100
Historically, clinical brain lesion studies in adults have provided evidence of functional specificity. Reference Broca101,Reference Wernicke102 Advanced magnetic resonance (MR)-based lesion analysis techniques are now available such as voxel-based lesion symptom mapping Reference Rorden, Karnath and Bonilha103 , lesion-behavior mapping Reference Rorden, Fridriksson and Karnath104,Reference Rorden and Karnath105 , lesion-network symptom-mapping, Reference Boes, Prasad and Liu106 and most recently, connectome-based lesion-symptom mapping. Reference Gleichgerrcht, Fridriksson, Rorden and Bonilha107 Despite relatively simple acquisition and processing leading to powerful structure–function comparisons, studies using structural imaging in perinatal stroke are somewhat scarce. Frontal lesions typically associate with cognitive impairments, Reference Crichton, Ditchfield and Gwini108 whereas damage to motor circuits (basal ganglia, M1) influence motor ability. Reference Ferre, Carmel, Flamand, Gordon and Friel109 Systematic lesion-symptom investigations in large numbers of perinatal stroke patients have not been reported thus far.
Volumetric analyses investigate differences in size of specific structures and the relation to clinical symptomology. Structures remote from, but connected to, the primary lesion have been shown to be altered by a process called diaschisis. Reference von Monakow110 Two recent studies in children with perinatal stroke have demonstrated smaller ipsilesional thalamic Reference Craig, Carlson and Kirton111 and contralesional cerebellar Reference Craig, Olsen and Mah112 volumes compared to the opposing hemisphere. These findings were highly related to motor and cognitive function supporting the clinical relevance of volumetric investigations in both lesioned and non-lesioned hemispheres. Cortical volumes above subcortical PVI lesions have also been shown to be smaller though were not found to be associated with motor dysfunction. Reference Li, Hodge, Wei and Kirton113 Volumetric studies thus provide converging evidence of neuroplastic changes occurring remotely from the original lesion that have clinical implications and may additionally shed light on changes in cortical volumes due to reorganization of function between hemispheres. More complex morphometry techniques investigating cortical thickness, gyrification, and sulcal depth quantify characteristics of gyri and sulci. Reference Luders, Thompson and Narr114 While both of these methods have yet to be utilized in perinatal stroke, results in pediatric populations are promising. Reference White, Su, Schmidt, Kao and Sapiro115,Reference Wierenga, Langen, Oranje and Durston116
In addition to morphometric characteristics, image intensity of T1- and T2-weighted images can quantify underlying tissue composition. Reference Oishi, Faria, Yoshida, Chang and Mori117,Reference Stüber, Morawski and Schäfer118 We have recently used this technique to explore potential alterations in myelination in perinatal stroke. Reference Yu, Carlson and Mineyko119 Myelination, as estimated by T1 intensity, was decreased in perilesional areas compared to distal, intrahemispheric areas, and a contralesional homolog which itself had decreased intensity compared to age-matched controls. Additional methods of assessing myelination include taking a ratio of signal intensities between T1- and T2-weighted images. Reference Glasser and Van Essen120,Reference Glasser, Goyal, Preuss, Raichle and Van Essen121 This method may be effective in discerning myelination processes in white matter and gray matter areas in the very preterm Reference Vandewouw, Young, Shroff, Taylor and Sled122 but has yet to be explored in perinatal stroke.
Diffusion Imaging
One of the most impactful neuroimaging sequences used in both research and clinical settings is diffusion-weighted MRI (dw-MRI). The high sensitivity and specificity of dw-MRI for acute cerebral infarction make it the gold standard for clinical diagnosis of acute perinatal stroke. Reference Lee, Mirsky and Beslow123–Reference Dudink, Mercuri and Al-Nakib125 The cytotoxic edema that results from infarction leads to high signal intensity on the dw-MRI and a corresponding decrease in apparent diffusion coefficient or so-called “restricted diffusion”. Reference Qiao, Malisza, Del Bigio and Tuor126–Reference Obenaus and Ashwal128 The clear visibility of acute infarcts on dw-MRI allows accurate diagnosis, early volume measurements, and informs clinical image scoring systems. Reference Beslow, Vossough and Dahmoush129 Additionally, infarct location on dw-MRI can be helpful in estimating both mechanisms and prognosis. Reference Mercuri, Barnett and Rutherford19,Reference Mercuri, Rutherford and Cowan24,Reference Rutherford, Counsell and Allsop130
Early diffusion imaging can also demonstrate changes in areas anatomically connected to, but remote from, the primary stroke lesion via diaschisis. The extent of diffusion restriction along the CST tracts has been correlated with long-term motor outcome. Reference Kirton, Domi, Shroff, Kouzmitcheva and deVeber15,Reference De Vries, Van der Grond, Van Haastert and Groenendaal131 Diffusion changes within specific locations may also be associated with functional outcomes, including visual and motor disability. Reference Dudink, Counsell, Lequin and Govaert132,Reference Koenraads, Porro and Braun133 Alterations in dw-MRI signal due to diaschisis is also measurable in highly connected structures remote from acute neonatal ischemic stroke, such as the thalamus and corpus callosum. Reference Srivastava, Rajapakse and Carlson134
More advanced diffusion imaging approaches may provide further insight into perinatal stroke plasticity. Diffusion tensor imaging (DTI) allows the estimation of diffusion properties within specific areas, structural tracts, and across the entire brain. Reference Basser, Pajevic, Pierpaoli, Duda and Aldroubi135,Reference Basser, Mattiello and LeBihan136 Using region of interest and other approaches, specific structural tracts can be modeled using DTI tractography. Fractional anisotropy (FA) describes the unity of diffusion into equal directions (isotropic and decreased FA) or in specific directions (anisotropic and higher FA). Additional diffusion metrics from DTI approaches include axial diffusivity and radial diffusivity of each metric describing different characteristics and possible damage to underlying white matter (WM) microstructure. Reference Basser and Jones137
White matter tract microstructure in children with perinatal stroke has been investigated using tractography, isolating specific tracts of interest and informing on sensorimotor network development following early brain injury. Differences in microstructure quantify potential alterations in underlying tract diffusion properties and structural connectivity. Lesioned side CST diffusion properties are often altered in perinatal stroke, the degree of which appears to correlate with motor deficits. Reference Hodge, Goodyear, Carlson, Wei and Kirton138 A large, controlled study correlated CST diffusion properties with detailed robotic measures of motor function in hemiparetic children with perinatal stroke demonstrated differences between arterial and venous stroke populations. Reference Kuczynski, Dukelow and Hodge139 A similar robotic approach capable of measuring complex sensory functions such as proprioception and kinesthesia demonstrated correlations between these clinical functions and the diffusion properties of the dorsal column medial lemniscus tract. Reference Kuczynski, Carlson and Lebel67 Future tractography approaches may explore broader components of sensorimotor (e.g., cortico-ponto-cerebellar) or more complex pathways mediating cognition. Further, tractography may also help to elucidate relative functional contributions of ipsilateral, contralateral, and interhemispheric pathways in models of developmental plasticity as described in the synaptic competition model above.
Tractography approaches can also be applied to the entire brain giving insight into global structural networks. Mathematical models such as graph theory can characterize pathways between regions and patterns of organization at the network level. Reference Fornito, Zalesky and Breakspear140 Such structural white matter connectome methods that have been applied to more heterogenous cerebral palsy populations suggest that more ordered structural connectivity correlates with clinical function. Reference Englander, Pizoli and Batrachenko141,Reference Ballester-Plané, Schmidt and Laporta-Hoyos142 Additionally, such structural connectome techniques may also quantify response to intervention, demonstrating improvements in clinical function following rehabilitation, Reference Englander, Sun and Case143 suggesting possible utility in investigating interventional plasticity. We have recently confirmed that a structural connectomics approach is feasible in perinatal stroke. Reference Craig, Hilderley and Kinney-Lang144 When investigating the non-lesioned hemisphere, we revealed modest but consistent relationships between baseline clinical motor function and some graph theory metrics. Reference Craig, Hilderley and Kinney-Lang144
Advanced diffusion imaging methods may therefore open the door for investigating how structural and whole-brain connectivity change following intensive occupational therapy or non-invasive brain stimulation. Baseline microstructural variables in the lesioned CST correlated with larger gains in rehabilitation in children with unilateral cerebral palsy Reference Manning, Fehlings and Mesterman145 though results vary. Reference Rickards, Sterling and Taub146 Larger sample sizes with more stringent inclusion and exclusion criterion and robust, high-dose interventions capable of effecting clinical and functional change are needed in order to better understand how different forms of therapy are reflected in neuroimaging outcomes of brain plasticity. An additional translational application would be improving the ability to predict individual responsiveness to such interventions and more personalized neurorehabilitation.
Development of novel white matter diffusion techniques may facilitate such progress. Neurite orientation dispersion and density imaging (NODDI) quantifies microstructural complexities of dendrites and axons in vivo by calculating neurite density index and orientation dispersion index. Reference Zhang, Schneider, Wheeler-Kingshott and Alexander147 These outcomes are sensitive to somas and glial cells that make up the extracellular space and the intraneurite space (between axons) thus quantifying angular variations of structure within a voxel characterizing white matter. NODDI has shown differences between lesioned and non-lesioned CSTs in children with unilateral cerebral palsy relating strongly to clinical function suggesting higher sensitivity for NODDI metrics (i.e., intracellular volume fraction) over traditionally used DTI metrics such as FA. Reference Nemanich, Mueller and Gillick148 Other emerging methods, such as myelin water fraction, quantitative inhomogeneous magnetization transfer, McDespot, and g-ratio, are all increasingly used in adults and may be translatable to pediatric populations. Reference Mackay, Whittall and Adler149–Reference Stikov, Campbell and Stroh152 These methods may allow further investigation of the developmental neuroplasticity of structural connections following perinatal stroke and how such connections change or explain clinical behavior.
Perfusion
Brain perfusion can be quantified using techniques such as arterial spin labeling (ASL) giving insight into cerebral blood flow (CBF) after stroke. Traditionally, perfusion techniques have been used in acute clinical diagnosis of stroke and can visualize potentially salvageable tissue and disruptions in blood flow such as hyper-, hypoperfusion, and the diffusion–perfusion mismatch all of which may inform subsequent treatments. Currently, there is a paucity of evidence as to how differences in blood perfusion persist into chronic stages post-perinatal stroke and how this may relate to long-term function.
ASL is a non-contrast MRI technique that magnetically “labels” a bolus of blood in the carotid arteries before it enters the brain. After a brief post-labeling delay, the labeled blood is detectible as it perfuses the gray and white matter, and whole-brain quantitative maps estimating CBF can be calculated. Disruptions in perfusion in the central and peripheral regions of the infarct and changes in these disruptions over time have been investigated in a few studies of perinatal AIS patients near birth shortly after experiencing seizures. Reference Wintermark and Warfield153–Reference Pienaar, Paldino and Madan156 Although potentially informative in acute cases, longer-term developmental implications of such perfusion disruptions remain unclear. Given its non-invasive nature (i.e., no use of radioactive tracers such as in single-photon emission computed tomography ), ASL may be informative in future studies to investigate chronic differences in brain perfusion and development of perfusion-based biomarkers correlated with function.
Functional MRI
Functional MRI (fMRI) is distinct from, but complementary to, structural, diffusion, and perfusion MR sequences. The basis of fMRI is the blood-oxygen-level-dependent (BOLD) contrast which reflects changes in regional fluctuations in deoxyhemoglobin as a surrogate marker for underlying neuronal activity. As neural activity increases in a given brain area, demand for oxygen also increases leading to increased CBF to the area. Such neural-vascular coupling results in a hemodynamic response causing changes in relative oxy- and deoxyhemoglobin concentrations. Fluctuations in BOLD response over time can be measured during a task or at rest, giving rise to powerful techniques for investigating localization of function as well as intrinsic functional connectivity among different brain regions.
Task-Based fMRI
Task-based fMRI is very effective in localizing brain areas involved in execution of specific tasks. Commonly, participants perform a task interleaved with periods of rest. BOLD signal fluctuations are then compared across task and rest time courses, and resulting statistical maps (contrasting periods of rest with task) show “active” areas of statistically significant task-synchronized changes in BOLD signal. These activation maps have high spatial resolution (at the mm level) for localization of areas of the brain actively recruited during specific tasks of interest and have been successful in characterizing patient-specific re-organization of functional areas after stroke in adults Reference Lake, Bazzigaluppi and Stefanovic157–Reference Rehme, Eickhoff, Rottschy, Fink and Grefkes159 and injuries to the developing brain. Reference Gaberova, Pacheva and Ivanov160
Resting-State fMRI
Low-frequency BOLD fluctuations can also be assessed in the absence of a task (resting-state fMRI [RS-fMRI]) having the distinct advantage of being task-free and thus accessible to participants who cannot successfully complete specific tasks. Participants typically fixate on a cross and think about “nothing in particular”. Since it is very unlikely for BOLD signal within multiple brain areas to significantly co-vary in time purely by chance, it is inferred that these areas are functionally connected. Reference Biswal, Yetkin, Haughton and Hyde161 Higher temporal correlations of BOLD response are thus taken to represent higher functional connectivity.
Multiple reliable resting state networks have been identified, Reference Grayson and Fair162 development and strengthening of such networks occurring across childhood and into early adulthood. The basic hubs for primary sensory functions (vision, audition, and sensorimotor) appear to develop very early, before birth. Reference van den Heuvel, Turk and Manning163 Throughout childhood, networks strengthen and follow a local-to-global pattern of development. Reference Fair, Cohen and Power164 Primary sensory networks appear first and are adult-like by around 8 years of age. Reference de Bie, Boersma and Adriaanse165 The higher-level executive, attention, and default mode networks follow later in childhood and appear to keep developing into the teenage years. Reference Grayson and Fair162
Analysis methods vary widely for RS-fMRI. Most commonly, methods assume that networks remain static in time. Seed-based analyses use regions of interest defined a priori based on anatomical atlases to define seeds for use in temporal cross-correlation measurements. Independent component analysis (ICA) is another commonly used data-driven method that parcellates significantly co-varying voxels into resting-state networks from multivariate BOLD signals without using regions of interest or atlases. Analyzing RS-fMRI as dynamic data is a more advanced emerging approach that has yet to be applied in perinatal stroke.
Sensorimotor Network
The hand sensorimotor system has been investigated via task fMRI during active or passive finger tapping or hand-squeezing tasks. In typically developing children and adults, the pattern of activation during a unimanual hand task usually involves contralateral primary sensorimotor, premotor, supplementary motor, and posterior parietal cortices as well as the contralateral thalamus and basal ganglia, and ipsilateral cerebellum. Though activations typically follow a contralateral pattern, lesser bilateral activations may occur. Reference Vandermeeren, Sebire and Grandin166–Reference Van de Winckel, Klingels and Bruyninckx168
After perinatal stroke, a comparison between paretic and less-affected hand fMRI activation patterns may show one of three main patterns of re-organization in M1 that appear consistent with synaptic competition models. Ipsilesional re-organization reflects recruitment of spared perilesional tissue to mediate sensorimotor tasks. Reference Staudt, Grodd and Gerloff42,Reference Thickbroom, Byrnes, Archer, Nagarajan and Mastaglia51,Reference Wilke, Staudt and Juenger169–Reference Gordon, Wood, Tournier and Hunt174 Contralesional re-organization results from the shifting (or preservation) of motor function in the paretic limb to contralesional (ipsilateral) sensorimotor areas which may be associated with more extensive damage to the injured hemisphere. Reference Staudt, Grodd and Gerloff42,Reference Thickbroom, Byrnes, Archer, Nagarajan and Mastaglia51,Reference Vandermeeren, Sebire and Grandin166–Reference Guzzetta, Bonanni and Biagi170,Reference Chu, Huttenlocher, Levin and Towle172 This reconfiguration results in ipsilateral cortex-mediating hand function (illustrated in Figure 2). More commonly, patients show a combination of both re-organization patterns (Figure 3) which results in bilateral representations of the paretic limb in both damaged and intact hemispheres. Reference Staudt, Grodd and Gerloff42,Reference Thickbroom, Byrnes, Archer, Nagarajan and Mastaglia51,166,168,171,173,175,176 Bilateral activations seen on fMRI may also be due to sensorimotor feedback from unintended mirror movements rather than bilateral representations of the sensorimotor network itself. Reference Staudt, Grodd and Gerloff42,Reference Vandermeeren, Sebire and Grandin166,167,Reference Weinstein, Green and Rudisch171,Reference Weinstein, Green and Geva177 More systematic investigation and online monitoring of mirror movements may give insight into clinical manifestations of sensorimotor re-organization.

Figure 3: Diffusion imaging. (A) Restriction of water diffusion caused by an acute ischemic infarction on this axial diffusion image appears bright leading to accurate clinical diagnosis as well as identification of secondary diaschisis displaced from the primary lesion (genu, splenium, thalamus, and basal ganglia). (B) Diffusion tractography can isolate white matter bundles of interest such as the cortical spinal tract using known anatomy (posterior limb of the internal capsule and cerebral peduncles [inlays]) to guide region of interest placement. (C) Whole-brain tractography can quantify structural connectivity of wider brain networks.
Evidence suggests that maintaining functional organization in ipsilesional areas is generally more effective in maximizing clinical motor function compared to the recruitment of contralateral or bilateral areas although considerable variability exists. Reference Staudt, Grodd and Gerloff42,Reference Rehme, Eickhoff, Rottschy, Fink and Grefkes159,Reference Chu, Huttenlocher, Levin and Towle172,Reference Weinstein, Green and Geva177 Further demonstrations using active (primarily motor) and passive (primarily sensory) unimanual tasks show that hemispheric dissociation of motor and sensory functions results in the motor representation re-organizing into the non-lesioned hemisphere and the sensory representations remaining in the lesioned hemisphere. Reference Staudt, Grodd and Gerloff42,Reference Thickbroom, Byrnes, Archer, Nagarajan and Mastaglia51,Reference Wilke, Staudt and Juenger169 Such organization may be maladaptive and could lead to decreased motor function – even if the somatosensory projections of the sensory pathways can successfully divert around the lesion in the damaged hemisphere to reach their original cortical targets. Reference Staudt, Braun and Gerloff178 Children who do have activations in the contralesional primary and secondary somatosensory cortices are likely to have poorer sensory function of their paretic hand. Reference Lemée, Chinier and Ali179 We do concede that for children with large lesions, ipsilesional reorganization may not be possible. In this situation, contralesional organization may be the only option and would not be maladaptive but rather necessary for preservation of residual function.
Many task fMRI studies investigate volume and peak height of activation areas during paretic hand sensorimotor tasks and have found that peaks are higher (i.e., higher statistical values) Reference Staudt, Grodd and Gerloff42,Reference Vandermeeren, Sebire and Grandin166,Reference Van de Winckel, Klingels and Bruyninckx168 and the volume of activations are larger (i.e., more suprathreshold voxels) Reference Vandermeeren, Sebire and Grandin166–Reference Van de Winckel, Klingels and Bruyninckx168 than for less-affected hand tasks or in comparison to controls. This commonly reported difference may be due to additional effort and wider cortical recruitment required to move the paretic hand. Height of activation peaks appear to be functionally relevant, Reference Vandermeeren, Sebire and Grandin166,Reference Guzzetta, Bonanni and Biagi170,Reference Weinstein, Green and Geva177 and peak heights in the ipsilesional central sulcus are highly correlated with motor function. Reference Weinstein, Green and Geva177 Ipsilateral parietal lobule and premotor cortex activations have also been linked to better performance on measures of dexterity in the paretic hand. Reference Vandermeeren, Sebire and Grandin166
Additional components of the sensorimotor network are likely altered and may include premotor, parietal, insular, superior temporal, and mesial frontal areas (supplementary and cingulate motor areas) as well as basal ganglia and cerebellum. Reference Staudt, Grodd and Gerloff42,Reference Vandermeeren, Sebire and Grandin166–Reference Van de Winckel, Klingels and Bruyninckx168,Reference Chu, Huttenlocher, Levin and Towle172,Reference van der Hoorn, Potgieser, Brouwer and de Jong175–Reference Weinstein, Green and Geva177 In typical development, basal ganglia activations are usually contralateral given the rich connectivity with many sensorimotor cortical areas. Reference Juenger, Grodd, Krägeloh-Mann and Staudt180 In stroke patients with a contralesional (ipsilateral) re-organization pattern, activations in the basal ganglia also show reorganization to the contralesional side. Reference Juenger, Grodd, Krägeloh-Mann and Staudt180 Interestingly, the cerebellum shows bilateral recruitment after perinatal stroke, which may be a compensatory response to an effortful task Reference Van de Winckel, Klingels and Bruyninckx168,Reference van der Hoorn, Potgieser, Brouwer and de Jong175 as exclusively ipsilateral recruitment is commonly seen in healthy adults. Reference Van de Winckel, Klingels and Bruyninckx168
Task fMRI can also be used to investigate interventional changes. CIMT-induced cortical reorganization may include a shift of motor control toward the lesioned hemisphere, but most studies have been in adult stroke. Reference Hodics, Cohen and Cramer181–Reference Liepert, Miltner and Bauder188 Small studies in HCP support possible alterations in lesioned M1 activity, Reference Juenger, Linder-Lucht and Walther45,Reference Walther, Juenger and Kuhnke46,Reference Sutcliffe, Gaetz, Logan, Cheyne and Fehlings189,Reference Juenger, Kuhnke and Braun190 with a predominant focus on M1 and limited exploration of network changes.
In typically developing children, resting-state sensorimotor networks are usually bilateral and symmetrical including somatosensory areas. ICA has demonstrated that children with AISs show trends toward lower sensorimotor system functional connectivity values compared to peers, but that children with PVI are not different from peers. Reference Ilves, Ilves and Laugesaar191 A small study of children with HCP revealed unilateral, asymmetrical sensorimotor networks, with notable differences compared to typically developing peers in contralesional motor and supplementary motor areas. Differences were not related to lesion volume. Reference Manning, Fehlings and Mesterman192 Children with more lateralized motor networks responded better to CIMT, indicating that RS-fMRI may be a useful correlate with intervention response. Reference Manning, Fehlings and Mesterman192 Other ICA studies have shown absent or disrupted resting-state networks Reference Papadelis, Ahtam and Nazarova193 in HCP compared to peers, but detectable networks were stronger than peers and possibly spatially expanded to include other nearby cortical areas. Reference Simon-Martinez, Jaspers and Alaerts194 A multimodal study in PVI suggested that differences in inter- and intrahemispheric functional connectivity may be CST-wiring specific. Reference Simon-Martinez, Jaspers and Alaerts194
Seed-based analyses have indicated that AISs have significantly lower interhemispheric functional connectivity values in sensorimotor cortical regions than peers Reference Qin, Sun and Zhang195,Reference Saunders, Carlson, Cortese, Goodyear and Kirton196 and that PVI show lower functional connectivity for supplementary motor areas compared to peers Reference Saunders, Carlson, Cortese, Goodyear and Kirton196 when seeding the lesioned M1. Higher intrahemispheric functional connectivity in the anterior supramarginal gyrus in the lesioned hemisphere of AIS was also observed compared to both typically developing peers and PVI. Reference Saunders, Carlson, Cortese, Goodyear and Kirton196 Alterations in resting-state sensorimotor networks within AIS and PVI groups did not seem to relate to motor function. Reference Ilves, Ilves and Laugesaar191,Reference Woodward, Gaxiola-Valdez, Goodyear and Federico197 In contrast, a recent study investigating PVI patients found functional connectivity within the sensorimotor network and the thalamus was associated with clinical motor and sensory function. Reference Woodward, Carlson and Kuczynski198
These RS-fMRI studies suggest that children with AIS have differences in network strength and topography compared to peers and that children with PVI seem to be more similar to peers. Relationships between functional connectivity and clinical motor/sensory function are inconsistent, possibly due to the heterogeneity of CST-wiring patterns. Future studies should continue to explore the role of the non-lesioned hemisphere as well as subcortical areas (i.e., thalamus and basal ganglia) as they may play an important role in sensorimotor system circuitry but have not been traditionally investigated in RS-fMRI studies.
Language Network
Uses of task fMRI and RS-fMRI are not limited to investigations of the sensorimotor network. The language network can be studied using fMRI tasks, such as verb generation, naming simple objects, saying words all starting with a certain letter, “word chains” in which the next word starts with the last letter of the preceding word, Reference Staudt, Lidzba and Grodd199 and story listening.
Language functions are significantly left hemisphere lateralized in most right-handed children, showing activations in anterior language areas such as left inferior frontal gyrus (i.e., Broca’s area) during language production tasks. During language perception tasks, activations are typically more posterior in left superior temporal gyrus (i.e., Wernicke’s area). This strong left laterality in typically developing participants develops over the course of development into early adulthood, with very young children usually showing bilateral organization. Reference Holland, Vannest and Mecoli200 Additional areas recruited during language tasks include the basal ganglia, intraparietal sulcus, mesial frontal cortex, anterior cingulate, and superior/middle frontal gyrus. Reference Staudt, Lidzba and Grodd199,Reference Jacola, Schapiro and Schmithorst201,Reference Tillema, Byars and Jacola202
Similar to the sensorimotor system, there is a significant amount of re-organization in the language system occurring after perinatal stroke that can be examined using task fMRI. The most common re-organization patterns for the language network following a left-sided stroke are bilateral Reference Staudt, Lidzba and Grodd199,Reference Jacola, Schapiro and Schmithorst201–Reference Szaflarski, Allendorfer and Byars207 or contralesional with remapping to homologous areas in the right hemisphere. Reference Staudt, Lidzba and Grodd199,Reference Jacola, Schapiro and Schmithorst201–Reference Raja Beharelle, Dick and Josse206,Reference Booth, Macwhinney and Thulborn208 Retention of function in left hemisphere perilesional areas is relatively uncommon and may preferentially occur in those children with smaller lesions remote from primary language areas. Reference Tillema, Byars and Jacola202,Reference Guzzetta, Pecini and Biagi203 There is considerable between-patient variability in these patterns suggesting that lesion location Reference Guzzetta, Pecini and Biagi203 and timing Reference Szaflarski, Allendorfer and Byars207 may play a role but apparently not lesion size. Reference Tillema, Byars and Jacola202,Reference Guzzetta, Pecini and Biagi203,Reference Raja Beharelle, Dick and Josse206,Reference Szaflarski, Allendorfer and Byars207
Language function in children with contralesional (right) or bilateral re-organization patterns is largely normal compared to peers, highlighting the potential for neuroplastic re-organization of extremely complex function after early injury. Reference Staudt, Lidzba and Grodd199,Reference Ilves, Tomberg and Kepler205,Reference Ballantyne, Spilkin, Hesselink and Trauner209 Children with bilateral activation patterns perform better than those with solely contra- or ipsilesional configurations. Reference Raja Beharelle, Dick and Josse206 The locations of contralesional (right) peak activations after perinatal stroke appear to be quite precise right hemisphere homologs (i.e., mirror images) of left hemisphere activations in typically developing peers, Reference Staudt, Lidzba and Grodd199,Reference Jacola, Schapiro and Schmithorst201–Reference Guzzetta, Pecini and Biagi203,Reference Ilves, Tomberg and Kepler205 with similar peak height Reference Staudt, Lidzba and Grodd199,Reference Tillema, Byars and Jacola202,Reference Guzzetta, Pecini and Biagi203 and activation volume. Reference Tillema, Byars and Jacola202 Aphasia in these children is not common if the injury was very early in life, yet observed language performance differences may relate to activation patterns. Reference Ilves, Tomberg and Kepler205
It is unclear whether it is the innate equipotentiality of left and right hemispheres at birth that makes the language network so plastic, Reference Staudt, Lidzba and Grodd199 whether bilateral re-organization reflects incomplete re-mapping of language function to the contralesional (right) hemisphere, Reference Jacola, Schapiro and Schmithorst201 or is possibly a disinhibition of “dormant circuitry” available after early injury to interhemispheric inhibitory mechanisms. Reference Tillema, Byars and Jacola202 Irrespective of the underlying mechanism, emerging evidence indicates that language function in children after perinatal stroke is largely normal compared to peers highlighting the potential for neuroplastic re-organization of extremely complex function after early injury.
Use of RS-fMRI to explore language networks has been limited in perinatal stroke. Overall, children with stroke may have a disruption of resting-state networks and weaker interhemispheric functional connectivity as compared to peers, with region-specific differences in connectivity that appears highly related to language performance. Reference Dick, Raja Beharelle, Solodkin and Small210,Reference Carlson, Sugden, Brooks and Kirton211
The bilateral symmetrical connectivity between left and right inferior frontal, superior temporal, and superior frontal gyri observed in typically developing children using seed-based analyses are not observed in children with left hemisphere stroke. Reference Carlson, Sugden, Brooks and Kirton211 These children have significant right laterality accompanied by lower functional connectivity in left inferior frontal gyrus, left middle temporal gyrus, left caudate nucleus, and left anterior thalamus. Reference Carlson, Sugden, Brooks and Kirton211 Intrahemispheric connectivity within the left hemisphere was highly correlated with language comprehension and verbal memory. Reference Carlson, Sugden, Brooks and Kirton211
During passive story listening, increased interhemispheric connectivity between right and left angular gyri was associated with poorer performance on a verbal comprehension test. Reference Dick, Raja Beharelle, Solodkin and Small210 By contrast, increased functional connectivity between right and left superior temporal gyri was associated with better performance on multiple measures of receptive language for children with perinatal stroke but typically developing children showed the opposite pattern. Cerebellar interhemispheric connectivity was not related to function.
Since language is a very complex skill, patients may have unique compensatory mechanisms. Resting-state networks in children are also still developing, and more global (i.e., longer range) connectivity has not yet developed. These factors may underlie differences in findings. More RS-fMRI investigations are called for to better understand the development of language resting-state networks after perinatal stroke.
Magnetic Resonance Spectroscopy
Magnetic resonance spectroscopy (MRS) quantifies neurometabolite concentrations within a brain region of interest (i.e., an MRS voxel). Since each neurochemical of interest has a different molecular structure, they resonate at different MR frequencies and can be individually identified within an MRS spectrum (Figure 5). MRS analysis involves statistical modeling of a line of best fit to the spectrum thus measuring neurometabolite concentration within the voxel. Measuring metabolite concentrations provides information on neuronal health (N-acetyl-aspartate [NAA]), cell membrane turnover (choline compounds [Cho] = [GPC + PCh]), energy metabolism (creatine compounds [Cre] = [Cr + PCr]), metabolic activity and excitatory neurotransmitters (glutamate/glutamine [Glx]), inhibitory neurotransmitters (γ-aminobutyric acid [GABA]), and health of glial cells (myo-Inositol [Ins]). Reference Rae212
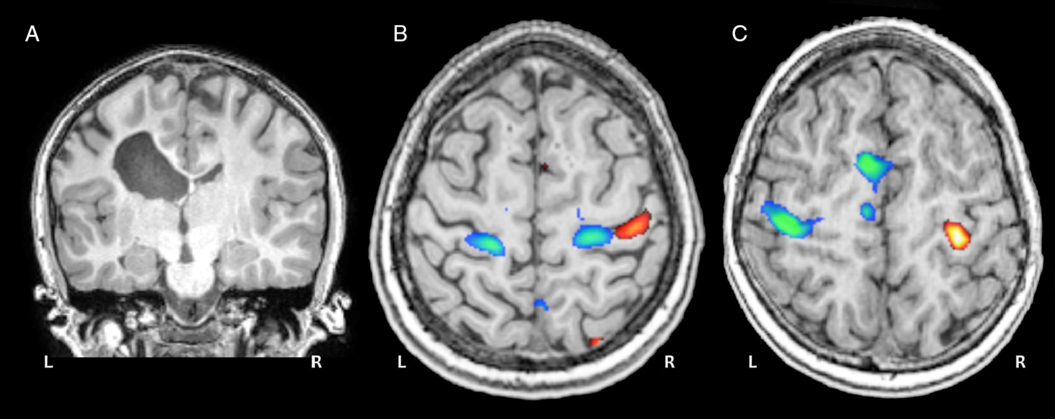
Figure 4: Motor task fMRI. (A) Coronal anatomical image of a 12-year-old child with a left periventricular venous infarction. (B) Task fMRI activation patterns during a ball squeeze task with the paretic (blue) and intact (red) hands show a bilateral re-organization pattern for the paretic hand. (C) Activation patterns for a typically developing control participant performing the same task with dominant (blue) and non-dominant (red) hands.

Figure 5: MR Spectroscopy. MRS voxel placements (yellow rectangles) guided by motor task fMRI activations for patients with (A) arterial ischemic stroke, (B) periventricular venous infarction, and (C) a typically developing control. (D) Resulting sample spectrum used to quantify neurometabolite concentrations within the MRS voxel reflecting underlying motor cortex neurochemistry. Adapted from Carlson et al., 2017.
MRS has not been widely used in perinatal stroke; however, meaningful comparisons have been made in the few studies to date. Lower concentrations of NAA, Cre, and Ins in the lesioned hemisphere motor cortex compared to the non-lesioned hemisphere were found in children with AIS. Reference Carlson, MacMaster, Harris and Kirton213 For children with PVI, hemispheric differences were found only for Cre. Children with spastic diplegia showed reductions in ratios of Cre, Cho, and Ins to NAA in basal ganglia which was related to age, gender, Reference Kulak, Sobaniec and Smigielska-Kuzia214 and severity of disability. Reference Kulak, Sobaniec, Kubas and Walecki215 This suggests that differences in current neuronal health underlie hemispheric differences after perinatal stroke rather than differences in glutamate-related metabolic activity or excitability. Further, NAA and Cre concentrations were highly positively related to motor function on multiple clinical tasks, Reference Carlson, MacMaster, Harris and Kirton213 suggesting that MRS neurometabolites are stable biomarkers of brain health after perinatal stroke.
Predictable changes in neurometabolite concentrations following interventions have been documented using MRS in children with perinatal stroke. Reliable reductions in excitatory metabolite concentrations (Glx, Cre) were seen under the electrode after inhibitory tDCS compared to sham stimulation. Reference Carlson, Ciechanski, Harris, MacMaster and Kirton216 Higher neurometabolite concentrations (NAA, Cho, Cre and Glx) at baseline were highly associated with better clinical motor function and a subset of these (Cre and Cho) were associated with subsequent response to intervention. Reference Carlson, Ciechanski, Harris, MacMaster and Kirton216 Another brain stimulation trial using excitatory stimulation found increases in Glx:Cre ratio in M1 accompanied by increased NAA:Cre, Cho:Cre, and Ins:Cre ratios in the basal ganglia and concurrent improvements in spasticity of the affected limb. Reference Auvichayapat, Aree-Uea and Auvichayapat217
In summary, the findings that neurometabolite concentrations are highly associated with clinical motor function seem promising for defining a stable biomarker of neurochemistry that is quantifiable, relate highly to function, and change predictably with interventions. MRS-derived metabolite concentrations may also shed light on excitatory and inhibitory neurotransmitter concentrations informing models of synaptic competition and neuroplasticity (described above) at the level of neurochemistry. Advances in imaging technology are required to shorten MRS sequences, making it feasible to sample more voxels across cortical and subcortical areas.
Challenges and Future Directions
While the existing body of literature in imaging of HCP is impressive given the unique challenges this population provides, we can see several major challenges for neuroimaging in this population that could be resolved moving forward.
First, neuroimaging and neurophysiology studies to date in HCP have been underpowered and likely too simplistic to map complex interventional and developmental changes in M1 and across the motor network. Collaborative efforts among researchers studying HCP cohorts could overcome the issue of small sample sizes with ongoing multi-site studies leading the way.
Second, cutting-edge imaging techniques are challenging to adapt to a pediatric population given possibly long acquisition durations and/or task requirements. Children with perinatal stroke often have physical and cognitive disabilities introducing additional challenges with compliance and increased head motion associated with tasks. As new innovative sequences are made more efficient and developments in MR hardware improve acquisition times, HCP imaging research will benefit.
Third, adult brain atlases are not ideal for use with pediatric brains and automated tissue segmentation, coregistration, and normalization algorithms often struggle with large lesions. As preprocessing pipelines improve, possibly incorporating powerful machine learning techniques, these challenges may become more manageable.
Fourth, neuroimaging in HCP has traditionally focussed on single modalities. Converging evidence from multi-modal studies reflects the power of neuroimaging and non-invasive brain stimulation. For example, combining measures of neurophysiology (i.e., TMS), WM tractography, and task fMRI would provide a more comprehensive understanding of the re-organized sensorimotor system after perinatal stroke. Reference Zewdie, Damji, Ciechanski, Seeger and Kirton37,Reference Staudt, Grodd and Gerloff42,Reference Weinstein, Green and Rudisch171
Fifth, neuroimaging analysis techniques often utilize correlation estimates as outcomes, but this risks misinterpretation as causative or predictive relationships despite best practice recommendations. Reference Poldrack, Huckins and Varoquaux218 It is important that such misinterpretations are guarded against and appropriately predictive models should be pursued such as regression that are better able to elucidate possible causality in identifying biomarkers for brain–behavior relationships.
Sixth, non-invasive brain stimulation techniques such as TMS and tDCS are promising given their tolerability and apparent effectiveness in modulating cortical excitability leading to lasting improvements in motor function. Given that pairing brain stimulation with intensive rehabilitation may have additive effects compared to either technique alone, new hardware developments could focus on portability and miniaturization to facilitate this pairing, including in younger children. A recent example is transcranial static magnetic stimulation which we recently demonstrated is well tolerated and may modulate motor learning in children. Reference Hollis, Zewdie and Nettel-Aguirre219 Further, customizing TMS/tDCS dosage for each patient based on their individualized brain anatomy Reference Gillick, Kirton, Carmel, Minhas and Bikson220–Reference Datta, Truong, Minhas, Parra and Bikson222 would effectively enhance personalized rehabilitation medicine.
Finally, as a field, we need to additionally focus not just on group statistics which may obscure important patient-specific nuances but move toward individualized patient neuroimaging biomarkers and comparisons with large normative databases of typically developing peers. By combining data from many imaging modalities and brain stimulation techniques, we can enhance our understanding of clinical function and neuroplasticity. Only by investigating individual imaging characteristics in each patient, we can use neuroimaging to enhance patient-centered interventional strategies.
Conclusion
Collectively, MRI modalities can be synergistically integrated to create comprehensive, in vivo models of brain structure and function to understand natural development and generate personalized maps of motor organization in children with perinatal stroke. Importantly, each method has also demonstrated the capacity to explore intervention-induced changes in brain function, bringing the potential to understand the mechanisms of therapy and neuromodulation in individual patients enhancing patient-centered personalized medicine after perinatal stroke.
Funding
BTC was supported by a Canadian Institute of Health Research (CIHR) Vanier Canadian Graduate Scholarship.
Program funding was provided by CIHR.
Statement of Authorship
BTC contributed to manuscript writing and editing. AH contributed to manuscript writing and editing. AK conceived and presented original idea and contributed to manuscript writing and editing. HLC contributed to manuscript writing and editing.