1. Introduction
Subduction of oceanic and/or continental crust into the mantle is one of the most significant processes that control the chemical evolution of the Earth and govern mantle dynamics (Hirose et al. Reference Hirose, Fei, Ma and Mao1999; Usui et al. Reference Usui, Nakamura, Kobayashi, Maruyama and Helmstaedt2003). To understand the fate of the subducting crust, i.e. the maximum depth of subduction or evolution and recycling of a subducting crust after slab break-off, especially during Precambrian time, it is essential to study the Precambrian eclogites that formed as a response to the increment of pressure during continued subduction. However, decoding the evolution of the Precambrian subducted crust is challenging owing to the paucity of orogenic/exhumed ultra-high-pressure (UHP) lithologies (eclogites and/or blueschists) in the pre-Mesoproterozoic geological record (e.g. Palin & Santosh, Reference Palin and Santosh2021). Kimberlites play an important role as tracers of Precambrian tectonics by directly sampling subduction-related eclogite xenoliths or inclusions in diamonds (e.g. Schmidberger et al. Reference Schmidberger, Simonetti, Heaman, Creaser and Whiteford2007; Shirey & Richardson, Reference Shirey and Richardson2011; Xu et al. Reference Xu, Kynicky, Tao, Liu, Zhang, Pohanka, Song and Fei2017). Thus, in the absence of orogenic/exhumed eclogites, such subduction-related eclogite xenoliths of pre-Mesoproterozoic age bear significant clues to decode the evolution of subducting crust and mantle dynamics (Jacob, Reference Jacob2004; Aulbach & Jacob, Reference Aulbach and Jacob2016).
The kimberlite-borne eclogite xenoliths, containing UHP minerals that correspond to varying pressures/depths of equilibration, have provided significant details on the evolution and recycling of subducting crust and the dynamics of the sub-continental lithospheric mantle beneath various cratons (e.g. Hills & Haggerty, Reference Hills and Haggerty1989; Smyth et al. Reference Smyth, Caporuscio and McCormick1989; Jacob et al. Reference Jacob, Schmickler and Schulze2003; Jacob, Reference Jacob2004; Shu et al. Reference Shu, Brey, Hoefer, Zhao and Pearson2016; Mikhailenko et al. Reference Mikhailenko, Aulbach, Korsakov, Golovin, Malygina, Gerdes, Stepanov and Xu2021). For example, an eclogite xenolith containing coesite suggests that the eclogite formed due to subduction (Jacob, Reference Jacob2004) at ≥2.8 GPa pressure (∼100 km depth) (Zhang & Zhang, Reference Zhang and Zhang2021), whereas the presence of K-rich clinopyroxene and supersilicic or majoritic garnet is indicative of pressure greater than 5 GPa (∼175 km depth) (Harlow & Veblen, Reference Harlow and Veblen1991; Van Roermund et al. Reference Van Roermund, Drury, Barnhoorn and De Ronde2000). In this study, we deployed in situ X-ray diffractometry (XRD), laser Raman spectroscopy and electron probe microanalysis (EPMA) to characterize coesite, majoritic garnet and supersilicic K-rich omphacite in one of the eclogite xenoliths from the Mesoproterozoic (∼1.1 Ga) Kalyandurg kimberlite of the Eastern Dharwar Craton, southern India. The geological implications for the presence of such UHP minerals are also discussed in the context of Precambrian mantle geodynamics. Furthermore, we also explore (i) the probable transport mechanism of a subducted slab within the upper mantle for the formation of such UHP minerals, and (ii) how they were brought back to the surface from the mantle transition zone during the Mesoproterozoic kimberlite eruption.
2. Geological framework
The Archaean Dharwar Craton of the southern Indian Shield is constituted of two distinct blocks, namely, the Western Dharwar Craton and the Eastern Dharwar Craton that differ on the basis of crustal thickness, lithological associations and grade of metamorphism (Gupta et al. Reference Gupta, Rai, Prakasam, Srinagesh, Bansal, Chadha, Priestley and Gaur2003; Ramakrishnan & Vaidyanadhan, Reference Ramakrishnan and Vaidyanadhan2010; Jayananda et al. Reference Jayananda, Santosh and Aadhiseshan2018). The Eastern Dharwar Craton is bounded between a steep mylonitic zone in the west, the Chitradurga Shear Zone, and the Proterozoic Eastern Ghats Mobile Belt in the east (Fig. 1a). The craton is a collage of granitoid plutons (Dharwar Batholith) and curvilinear greenstone belts that accreted obliquely to the Western Dharwar Craton during the Neoarchaean (Chadwick et al. Reference Chadwick, Vasudev and Hegde2000). The continental crust of the craton is considered to have evolved by accretion during Archaean time in five major episodes (∼3.45–3.33 Ga, ∼3.23–3.15 Ga, ∼3–2.96 Ga, ∼2.7–2.6 Ga and ∼2.56–2.5 Ga), similar to the other cratons of the globe (Jayananda et al. Reference Jayananda, Aadhiseshan, Kusiak, Wilde, Sekhamo, Guitreau, Santosh and Gireesh2020).

Fig. 1. (a) Generalized geological map of peninsular India. (b) Geological map of the Wajrakarur Kimberlite Field in the Eastern Dharwar Craton, India (modified after Nayak & Kudari, Reference Nayak and Kudari1999; Shaikh et al. Reference Shaikh, Patel, Ravi, Behera and Pruseth2017).
Numerous kimberlite pipes are known from the Dharwar Craton and are grouped into the Narayanpet kimberlite field (NKF) in the north, Raichur kimberlite field (RKF) and Tungabhadra kimberlite field (TKF) in the centre and Wajrakarur kimberlite field (WKF) in the south (Fig. 1b). These kimberlites are either of Mesoproterozoic age (∼1.1 Ga; Kumar et al. Reference Kumar, Heaman and Manikyamba2007; Chalapathi Rao et al. Reference Chalapathi Rao, Creaser, Lehmann and Panwar2013; Pandey & Chalapathi Rao, Reference Pandey and Chalapathi Rao2020; Dongre et al. Reference Dongre, Lavhale and Li2021) or of Late Cretaceous age (∼90 Ma; Chalapathi Rao et al. Reference Chalapathi Rao, Dongre, Wu and Lehmann2016). The ∼1.1 Ga old WKF is the largest of all these fields and is constituted of different kimberlite clusters: the Wajrakarur, Lattavaram, Chigicherla, Kalyandurg, Timmasamudram and Gooty (Fig. 1b). The oval-shaped kimberlite intrudes the Closepet Granite, which is considered to be a manifestation of late Neoarchaean (∼2.5 Ga; Friend & Nutman, Reference Friend and Nutman1991) tectonomagmatic activity in the Eastern Dharwar Craton. The present study deals with an eclogite xenolith found in pipe KL-2 of the Kalyandurg cluster, which hosts the largest number of eclogite xenoliths, unlike the other kimberlite pipes of the WKF that are dominated by peridotite xenoliths (Nehru & Reddy, Reference Nehru and Reddy1989).
The eclogite xenoliths from pipe KL-2 were studied in detail by Patel et al. (Reference Patel, Ravi, Thakur, Rao and Subbarao2006, Reference Patel, Ravi, Anil Kumar, Naik, Thakur, Pati and Nayak2009) and Dongre et al. (Reference Dongre, Jacob and Stern2015) in terms of their mineralogy, texture and pressure–temperature (P–T) evolution. Based on the mineralogy, Patel et al. (Reference Patel, Ravi, Thakur, Rao and Subbarao2006, Reference Patel, Ravi, Anil Kumar, Naik, Thakur, Pati and Nayak2009) classified the eclogites from pipe KL-2 as bimineralic (garnet + omphacite ± rutile), enstatite-bearing (garnet + omphacite + enstatite ± rutile) and celsian-bearing kyanite eclogites (garnet + omphacite + kyanite + celsian ± rutile). In these reports, they inferred the presence of former supersilicic/majoritic garnet. Haggerty & Birkett (Reference Haggerty and Birkett2004) and Babu et al. (Reference Babu, Griffin, Panda, O’Reilly and Bhaskar Rao2008) additionally mentioned the presence of coesite in the eclogites of pipe KL-2. However, the proper identification and undisputed characterization of coesite and majoritic garnet were not established by the previous workers. The P–T estimations by Patel et al. (Reference Patel, Ravi, Thakur, Rao and Subbarao2006, Reference Patel, Ravi, Anil Kumar, Naik, Thakur, Pati and Nayak2009) range between 2.8–5 GPa and 800–1225 °C, whereas Dongre et al. (Reference Dongre, Jacob and Stern2015) calculated the P–T as 4.5–5.3 GPa and 1060–1220 °C, which translates to a minimum lithospheric thickness of ∼150 km if lithostatic stress is taken into account. Oxygen isotope signatures retrieved from the garnet of eclogites of pipe KL-2 strongly suggest that these eclogites formed owing to subduction (Dongre et al. Reference Dongre, Jacob and Stern2015).
3. Analytical methods
The in situ XRD analysis was carried out at the Inter University Accelerator Centre (IUAC), New Delhi, using a PANalytical EMPYREAN X-ray diffractometer with CuKɑ radiation (λ = 1.5406 Å) functioning at 45 kV and 40 mA to identify the minerals from thin-sections. The studied thin-section was scanned at a 2θ range of 15° to 50° with a step size of 0.02 µm and a count time of 1 second per step. The identified minerals were confirmed by their diffraction patterns from the powder diffraction database of the International Centre for Diffraction Data (ICDD).
Laser Raman analysis and Raman intensity mapping were carried out using a Horiba Jobin Yvon LabRAM HR laser Raman microprobe in the Raman and Fluid Inclusion Laboratory at Wadia Institute of Himalayan Geology, Dehradun. The instrument has a spectral resolution of <1 cm−1. In the present study, a 514 nm laser of argon ion (Ar+) source was used. Standard silicon was used for calibration, which shows a Raman shift spectrum at 520.59 cm–1. Calibration was performed with an error of 0.1 cm–1. Accumulations were done for 2 seconds, while the acquisition time was set for 7 to 6 seconds. The grating was fixed at 600 grooves/mm, while the hole width was set as 400 µm. All the measurements were performed under a ×100 objective lens. Repeated spectra were recorded in the 200–700 cm−1 region to obtain better signals for silica polymorphs.
A Horiba Jobin Yvon LabRAM HR Evolution spectrometer installed at the Department of Chemistry, Banaras Hindu University, was also used for laser Raman analysis. The sample was irradiated with the 532 nm laser. Standard silicon was used for calibration, which shows a Raman shift spectrum at 520.8 cm–1. Accumulations were carried out for 4 to 10 seconds, and the acquisition time was set for 7 to 15 seconds. The grating was fixed at 1800 grooves/mm, while the hole width was set as 400 µm. All the measurements were performed under a ×100 objective lens. Repeated spectra were recorded in the 200–700 cm−1 region to obtain better signals for quartz and coesite.
Various mineral phases present in the studied xenolith were analysed using a CAMECA-SXFive electron probe microanalyser at the Department of Geology, Banaras Hindu University. Polished thin-sections were coated with a 20 nm carbon layer using a LEICA-EM ACE200 carbon coater prior to the analysis. The instrument was operational at an accelerating voltage of 15 kV and 10 nA current from a LaB6 filament source. Wavelength dispersive X-ray spectrometry in combination with LIF, PET, LPET, TAP and LTAP crystals were used for the quantitative analyses. The diameter of the beam and peak time throughout the analysis were ∼1 μm and 10 s, respectively. X-ray intensities were calculated by the X-PHI correction method. Various synthetic and natural reference materials provided by CAMECA-AMETEK were utilized during the calibration.
4. Results
4.a. Petrography
A sample from the eclogite xenolith with variable size (6 × 4 cm to 1 × 1 cm) from the KL-2 kimberlite pipe consists of pink-coloured, coarse-grained and rounded to sub-rounded garnet grains (∼400–800 µm in diameter) set in a greyish green to white matrix of altered omphacite. In addition, fine-grained, irregular-shaped majoritic garnet (1000 × 100 µm), kyanite (1200 × 400 µm), quartz/coesite (∼50–200 µm in diameter) and rutile were also observed as discrete grains with accessory apatite, barite and chalcopyrite. The whitish appearance of the xenolith is either due to the late-stage alteration or because of interaction between the xenolith and the host kimberlite during ascent (Fig. 2a, b). Coarse-grained garnet is in textural equilibrium with kyanite and quartz/coesite (Fig. 3a, b). These garnet grains are often rimmed by kelyphitic intergrowth of Ca–Al silicate and K-feldspar (Figs 3a, b, 4a, b). Omphacite is fractured and altered. Pristine omphacite compositions are exclusively preserved in the core of altered grains (Fig. 3c). The fine-grained and anisotropic majoritic garnet exclusively occurs along the periphery of the omphacite grains, separating the omphacite from the kelyphitic rim of garnet (Figs 3d, 4c, d). Elongated kyanite grains contain hydrous Ca–Al silicates along the fractures. Quartz/coesite occurs in close association with garnet in the matrix and is often rimmed by polycrystalline quartz (Fig. 4a, b). A curved intragranular deformation fabric is commonly observed within the quartz/coesite (Fig. 3e, f). The quartz/coesite grains are also bleached along the cracks that propagate from the altered matrix similar to that exhibited by omphacite (Figs 3e, f, 4a, b). Rutile is rounded to semi-rounded in shape and often rimmed by ilmenite. Occasionally, rutile is found within the necklace texture that formed at the interface between coarse-grained garnet and omphacite.
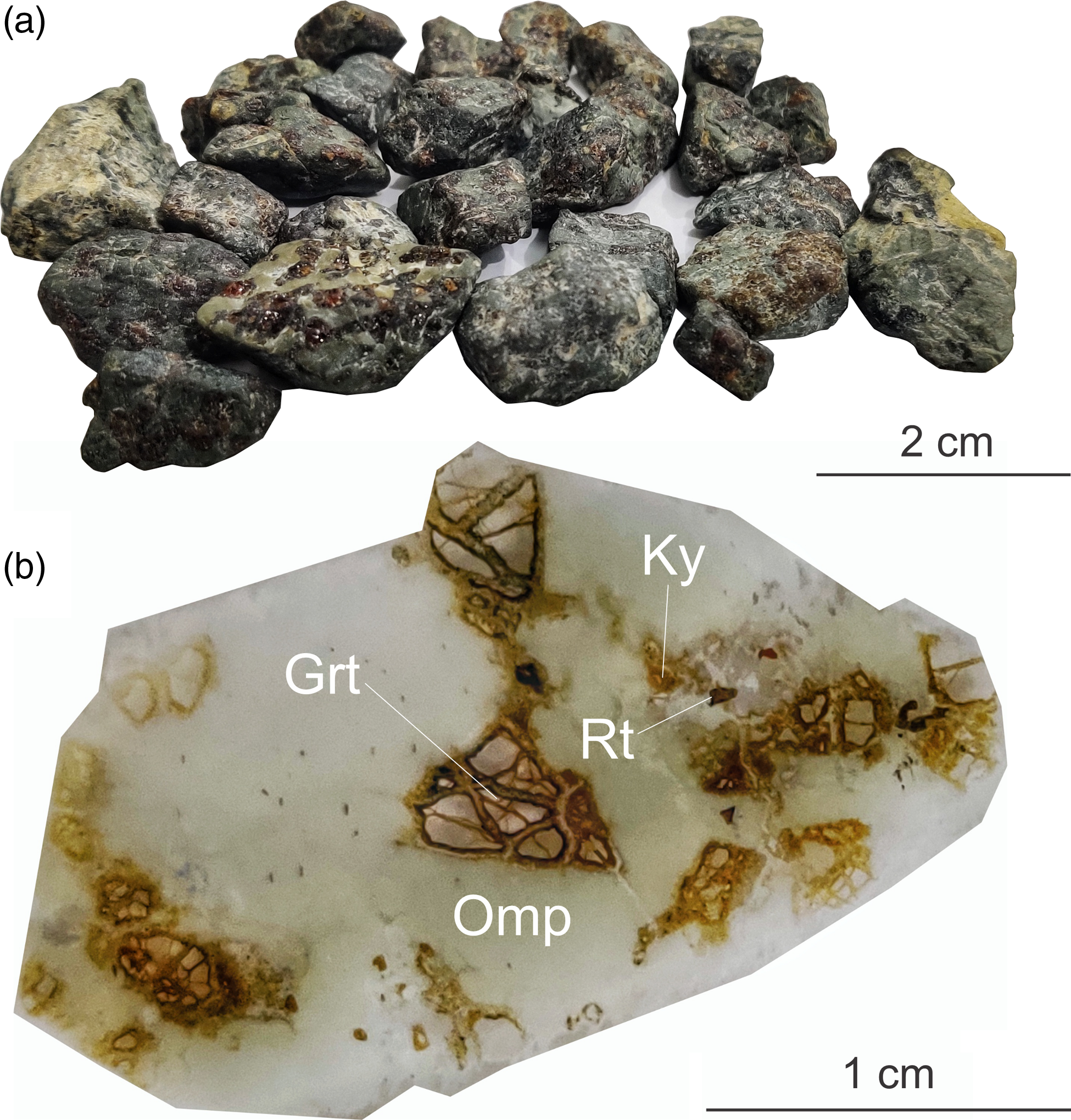
Fig. 2. (a) Hand specimens/nodules of eclogite xenoliths collected from the KL-2 kimberlite pipe of the Kalyandurg cluster. (b) Scanned thin-section of the coesite-bearing eclogite sample. Mineral abbreviations: Grt – garnet; Ky – kyanite; Rt – rutile; Omp – omphacite.

Fig. 3. Back-scattered electron (BSE) images of the studied sample depicting (a, b) textural equilibrium of coesite with garnet and kyanite, (c) necklace texture formed at the interface between garnet and clinopyroxene. Fresh omphacite is observed in the core of the altered clinopyroxene. BSE images of (d) the majoritic garnet along the periphery of omphacite, (e, f) different coesite grains found in the matrix. Please note the intragranular fractures within the grains. Mineral abbreviations: Coe – coesite; Grt – garnet; Ky – kyanite; Maj – majoritic garnet; Omp – omphacite.
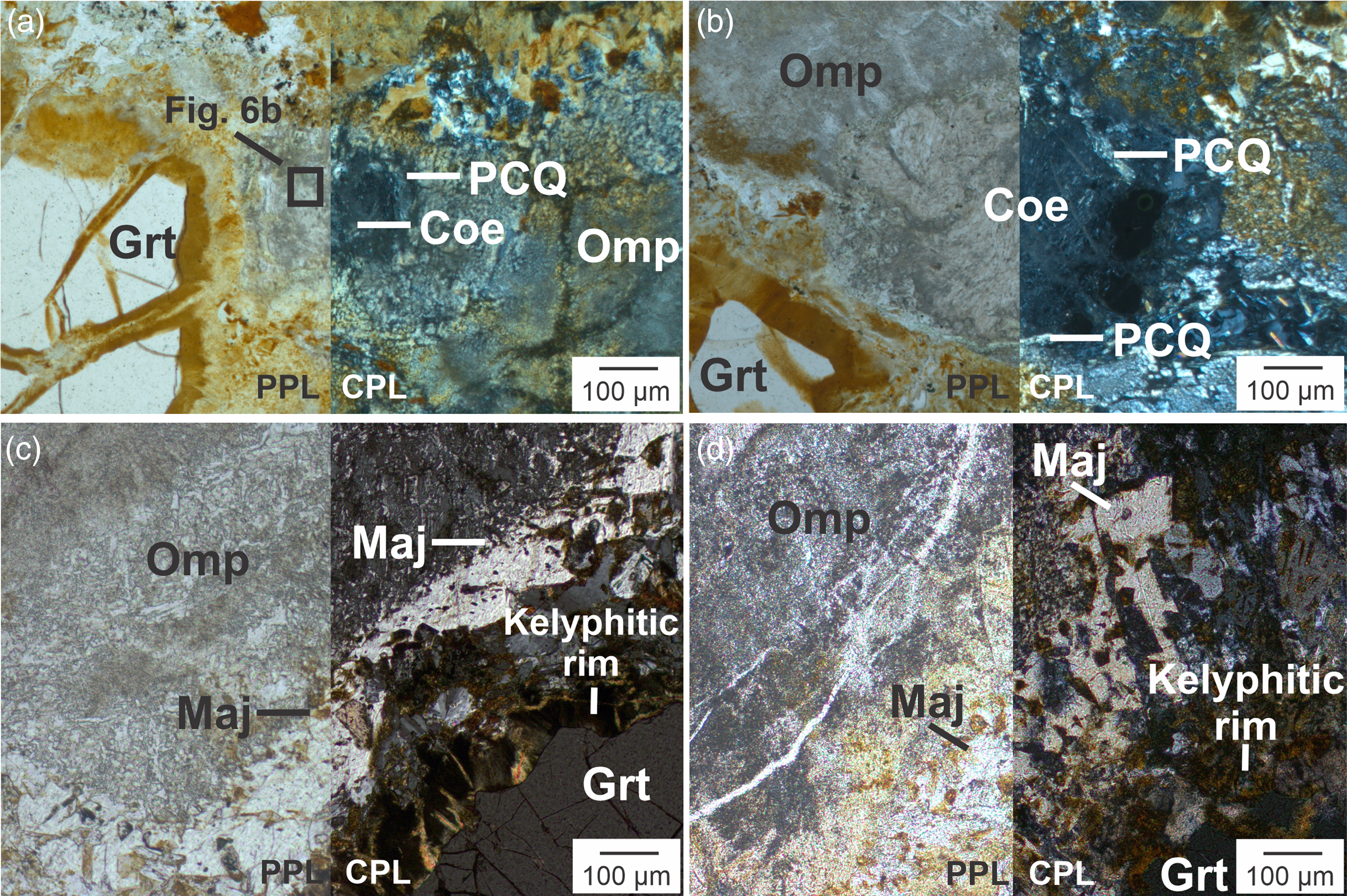
Fig. 4. (a, b) Plane- (PPL) and cross-polarized (CPL) photomicrographs showing coesite (Coe) rimmed by polycrystalline quartz (PCQ), along with omphacite (Omp) and garnet (Grt). The black rectangle in (a) marks the location of the Raman intensity map that is shown in Figure 6b. (c, d) PPL and CPL photomicrographs showing the presence of majoritic garnet along the periphery of omphacite. Please note that the majoritic garnet is not isotropic under CPL.
4.b. Characterization of coesite
Even though the mention of coesite from the eclogites of pipe KL-2 was made before (see Haggerty & Birkett, Reference Haggerty and Birkett2004; Babu et al. Reference Babu, Griffin, Panda, O’Reilly and Bhaskar Rao2008), no unequivocal supporting evidence from quantitative analytical techniques was provided. Here, a combination of scanning electron microscopy – energy-dispersive X-ray spectroscopy (SEM-EDS), in situ XRD and laser Raman spectroscopy coupled with Raman intensity mapping were carried out to characterize the coesite. The initial evidence in favour of quartz/coesite was obtained from SEM-EDS spectra from several grains. A representative EDS spectrum of pure SiO2 is provided in the inset of Figure 5. To distinguish between quartz and coesite, several transects were chosen across the thin-sections for in situ XRD analysis based on the location of quartz/coesite in the sample. The acquired spectra exhibit a collective XRD pattern of all the minerals present. From a representative transect, two characteristic peaks of quartz at 4.197 Å (faint peak with relative intensity = 20.6 %) and 3.302 Å (sharp peak with relative intensity = 100 %; Fig. 5) were identified. The presence of coesite, on the other hand, was confirmed by its nine characteristic peaks (Fig. 5). The characteristic peaks of coesite with higher relative intensity at 3.262 Å (relative intensity = 21.4 %), 2.992 Å (relative intensity = 100 %), 2.942 Å (relative intensity = 19.4 %) and 2.893 Å (relative intensity = 5.1 %) are strong and prominent. Additionally, five characteristic peaks of coesite with lower relative intensity at 5.212 Å (relative intensity = 0.1 %), 4.237 Å (relative intensity = 0.1 %), 2.678 Å (relative intensity = 6.4 %), 2.623 Å (relative intensity = 4.5 %) and 2.073 Å (relative intensity = 1 %) are faint and masked by the background owing to the presence of multiple strong peaks in the vicinity. The characteristic peaks of garnet, kyanite, omphacite and rutile (TiO2-I), are also marked in the collective XRD spectra obtained from the representative traverse (Fig. 5).

Fig. 5. Representative in situ XRD spectra of the entire thin-section. The red and blue colour bands are coesite and quartz, respectively. The characteristic XRD spectra of the essential minerals are also marked. The inset figure shows the representative SEM-EDS spectrum of quartz/coesite.
The characteristic Raman shift spectra of both quartz and coesite were obtained from the unaltered/pristine parts of the targeted grains. The representative monomineralic coesite was identified by the presence of a characteristic sharp peak at 522.3 cm−1, with subordinate coesite peaks at 388.05 cm−1 and 321.1 cm−1 (Fig. 6a). The presence of monomineralic coesite is further confirmed by Raman intensity mapping. The Raman intensity map for coesite at the 520.8 cm−1 Raman shift position revealed a strong presence of monomineralic coesite clusters within the representative grain (Figs 4a, 6b). The representative bimineralic quartz and coesite were characterized by a sharp and symmetric Raman shift spectra at 462.7 cm−1 (quartz) and 518.4 cm−1 (coesite), with minor peaks of quartz and coesite at 355.5 cm−1 and 271.0 cm−1, respectively (Fig. 6a). A strong representative Raman shift peak at 464.2 cm−1 with a subordinate peak at 356.5 cm−1 confirmed the presence of monomineralic quartz (Fig. 6a) that occurs mostly along the rim of the grain. Occasionally, patches of bimineralic quartz + coesite and monomineralic quartz are randomly distributed adjacent to the monomineralic coesite, without forming any core–mantle–rim structure of coesite, quartz + coesite and quartz.

Fig. 6. (a) Representative laser Raman spectra of the monomineralic coesite (522.3, 388.05 and 321.1 cm−1; red), bimineralic quartz + coesite (quartz: 462.7 and 355.5 cm−1; coesite: 518.4 and 271 cm−1; purple) and monomineralic quartz (464.2 and 356.5 cm−1; blue), respectively. The standard spectra of quartz and coesite from the Rruff database is also provided for reference. (b) Representative Raman intensity map of a coesite/quartz grain showing the strong presence of coesite as a cluster.
4.c. Characterization of coarse-grained and majoritic garnet
The composition of the coarse-grained and rounded to sub-rounded garnet cores is represented by Alm38–41Prp14–15Grs44–47Sps0–1 with XFe ranging between 0.72 and 0.74 (Table 1). The Si atoms per formula unit (apfu) range from 2.90 to 3.02 (Table 1; Fig. 7). Except for one point with Si apfu of 3.02, such garnet grains are completely devoid of Na (Table 1). There is another group of compositionally and texturally distinct garnet with high silica content (Si apfu = 3.18–3.61) (Table 2; Fig. 7). The Na2O and CaO contents in these fine-grained and irregular-shaped garnets vary from 0.48 to 1.60 wt % and 19.64 to 21.47 wt %, respectively (Table 2), which is higher than that in the garnet with Si apfu 2.90–3.02. Considering the Si apfu ≥3.05 as a threshold value for the characterization of supersilicic or majoritic garnet (Tappert et al. Reference Tappert, Stachel, Harris, Muehlenbachs, Ludwig and Brey2005), these high Si–Na–Ca garnet are classified as majoritic garnets (Fig. 7).
Table 1. EPMA mineral chemical data of garnet core

n.d = not detected.
XPrp = (Mg/(Fe2+ + Mg + Ca + Mn)), XAlm = (Fe2+/(Fe2+ + Mg + Ca + Mn)), XGrs = (Ca/(Fe2+ + Mg + Ca + Mn)), XSps = (Mn/(Fe2+ + Mg + Ca + Mn)).
Peak Ta = 1579–1119 °C, Peak Tb = 1478–1085 °C.
Peak Pa = 8–5 GPa, Peak Pc = 7–5 GPa.
a = using Krogh Ravna & Terry (Reference Krogh Ravna and Terry2004).
b = using Ellis & Green (Reference Ellis and Green1979).
c = P calculated using T estimated by Ellis & Green (Reference Ellis and Green1979) projected on the steady-state geotherm of the Kalyandurg cluster taken from Karmalkar et al. (Reference Karmalkar, Duraiswami, Chalapathi Rao and Paul2009).

Fig. 7. A Si apfu versus Mg apfu plot of the garnet and majoritic garnet from the studied sample. The Si = 3.05 line demarcates the coarse-grained garnet from majoritic garnet.
Table 2. EPMA mineral chemical data of majoritic garnet

Pressure estimated using the following barometers: 1 – Tao et al. (Reference Tao, Fei, Bullock, Xu and Zhang2018); 2 – Collerson et al. (Reference Collerson, Williams, Kamber, Omori, Arai and Ohtani2010); 3 – Wijbrans et al. (Reference Wijbrans, Rohrbach and Klemme2016); 4 – Beyer & Frost (Reference Beyer and Frost2017).
Evidence in support of majoritic garnet comes from the laser Raman shift spectra (Fig. 8). The broad peaks between 800 and 900 cm−1 obtained from inclusion-free majoritic garnet are the characteristic Raman shift spectra for majoritic garnet (Fig. 8), which indicate the presence of excess Si in the octahedral site (Gillet et al. Reference Gillet, Sautter, Harris, Reynard, Harte and Kunz2002; Kunz et al. Reference Kunz, Gillet, Fiquet, Sautter, Graafsma, Conrad and Harris2002), along with the characteristic sharp peaks at 911.5 and 913.2 cm−1 (Kunz et al. Reference Kunz, Gillet, Fiquet, Sautter, Graafsma, Conrad and Harris2002; Stähle et al. Reference Stähle, Altherr, Nasdala and Ludwig2011). Compared with the global dataset of majoritic garnet, the composition of the majoritic garnet in this study is unique in terms of high Ca and low Mg content. Hence, it is difficult to compare the obtained Raman shift spectra with published data. However, we compared our Raman shift spectra with the Raman shift spectra of Hofmeister et al. (Reference Hofmeister, Giesting, Wopenka, Gwanmesia and Jolliff2004) and Stähle et al. (Reference Stähle, Altherr, Nasdala and Ludwig2011) because of the nearly identical Si apfu. The Raman shift spectra at 549.1, 566.5, 661.8 and 663.6 cm−1 for majoritic garnet with Si apfu 3.39 of Hofmeister et al. (Reference Hofmeister, Giesting, Wopenka, Gwanmesia and Jolliff2004) and Stähle et al. (Reference Stähle, Altherr, Nasdala and Ludwig2011) are matched with the Raman shift spectra obtained in this study. The subordinate peaks at 347.8, 379.9, 549.1, 1013 and 1014.6 cm−1 correspond well with the Raman spectra of the omphacite (Fig. 8).

Fig. 8. Representative laser Raman spectra of the majoritic garnet (red) along with the standard Raman spectra of omphacite (Rruff database) and majoritic garnet of Hofmeister et al. (Reference Hofmeister, Giesting, Wopenka, Gwanmesia and Jolliff2004) and Stähle et al. (Reference Stähle, Altherr, Nasdala and Ludwig2011), which have identical Si apfu compared to the studied sample.
4.d. Mineral chemistry of K-omphacite
Since the clinopyroxenes are highly altered, the composition of their rims could not be determined. The cores of clinopyroxene, which are devoid of any intergrowth/inclusions (Fig. 3c), are omphacitic with Na2O ranging between 3.97 and 5.87 wt % (Table 3). Interestingly, these omphacites are supersilicic (Si >2 apfu; Table 3) with a negligible Ca-Tschermakite component. In addition, the omphacites are rich in Al2O3 (16.98–19.53 wt %) and K2O (0.73–2.94 wt %, average = 1.78 ± 0.21 wt %) (Table 3; Fig. 9).
Table 3. EPMA mineral chemical data of K-omphacite


Fig. 9. K2O-in-Cpx versus Al2O3-in-Cpx plot of various diamond-hosted clinopyroxene found in the xenoliths. Please note that the clinopyroxene of this study contains one of the highest amounts of Al2O3 and K2O. Data sources are: 1 – Jaques et al. (Reference Jaques, O’Neill, Smith, Moon and Chappell1990); 2 – Snyder et al. (Reference Snyder, Taylor, Crozaz, Halliday, Beard, Sobolev and Sobolev1997); 3 – Harlow (Reference Harlow1997); 4 – Prinz et al. (Reference Prinz, Manson, Hlava and Keil1975); 5 – Pokhilenko et al. (Reference Pokhilenko, Sobolev, Reutsky, Hall and Taylor2004); 6 – Kaminsky et al. (Reference Kaminsky, Zakharchenko, Griffin, Channer and Khachatryan-Blinova2000); 7 – Stachel et al. (Reference Stachel, Brey and Harris2000); 8 – Bindi et al. (Reference Bindi, Safonov, Yapaskurt, Perchuk and Menchetti2003).
4.e. Geothermobarometry
Two conventional geothermobarometers were used to constrain the maximum P–T conditions under which the coarse-grained garnet–clinopyroxene–kyanite–rutile–coesite assemblage evolved. Sobolev et al. (Reference Sobolev, McCammon, Taylor, Snyder and Sobolev1999) observed that Fe3+/total Fe has a compensation effect on garnet and clinopyroxene, which, in turn, does not change actual temperature estimates of the eclogites. Thus, the Fe2+ of garnet and clinopyroxene was considered as total Fe for the Mg–Fe2+ exchange geothermometer of Ellis & Green (Reference Ellis and Green1979). On the other hand, the garnet–clinopyroxene–kyanite–coesite ± phengite net-transfer geothermobarometer of Krogh Ravna & Terry (Reference Krogh Ravna and Terry2004) is not effected by the Fe3+/total Fe estimation (Krogh Ravna & Paquin, Reference Krogh Ravna and Paquin2003). Owing to the presence of excess silica (>2 apfu), the AlIV in T site could not be calculated (AlIV = 2-Si) for clinopyroxene, which barred the use of the garnet–clinopyroxene geobarometer of Beyer et al. (Reference Beyer, Frost and Miyajima2015).
Application of the qualitative K-in-pyroxene barometer of Safonov et al. (Reference Safonov, Perchuk and Litvin2005) estimates a pressure of between 6 and 7 GPa (Fig. 10). The core compositions of coarse-grained garnet and clinopyroxene were used for the garnet–clinopyroxene geothermometer of Ellis & Green (Reference Ellis and Green1979). The temperature estimation ranges between 1085 and 1478 °C (Table 1). Considering the temperature range, the pressure is calculated as ∼5–7 GPa by projecting the temperature on the steady-state geotherm of the Kalyandurg cluster during the xenolith entrainment in Mesoproterozoic time (reviewed in Karmalkar et al. Reference Karmalkar, Duraiswami, Chalapathi Rao and Paul2009). The projected pressure is higher than the value of Patel et al. (Reference Patel, Ravi, Thakur, Rao and Subbarao2006, Reference Patel, Ravi, Anil Kumar, Naik, Thakur, Pati and Nayak2009) and Dongre et al. (Reference Dongre, Jacob and Stern2015) owing to the increment of temperature estimation. The unambiguous characterization of coesite for the first time from the Kalyandurg cluster enabled us to use the garnet–clinopyroxene–kyanite–coesite ± phengite geothermobarometer of Krogh Ravna & Terry (Reference Krogh Ravna and Terry2004). The temperature estimation ranged between 1119 and 1579 °C, whereas the pressure was computed as ∼5–8 GPa (Fig. 11a; Table 1).

Fig. 10. K-in-Cpx versus Na-in-Cpx plot (after Safonov et al. Reference Safonov, Perchuk and Litvin2005) showing that the pressure of our sample is mostly constrained between 6 and 7 GPa.
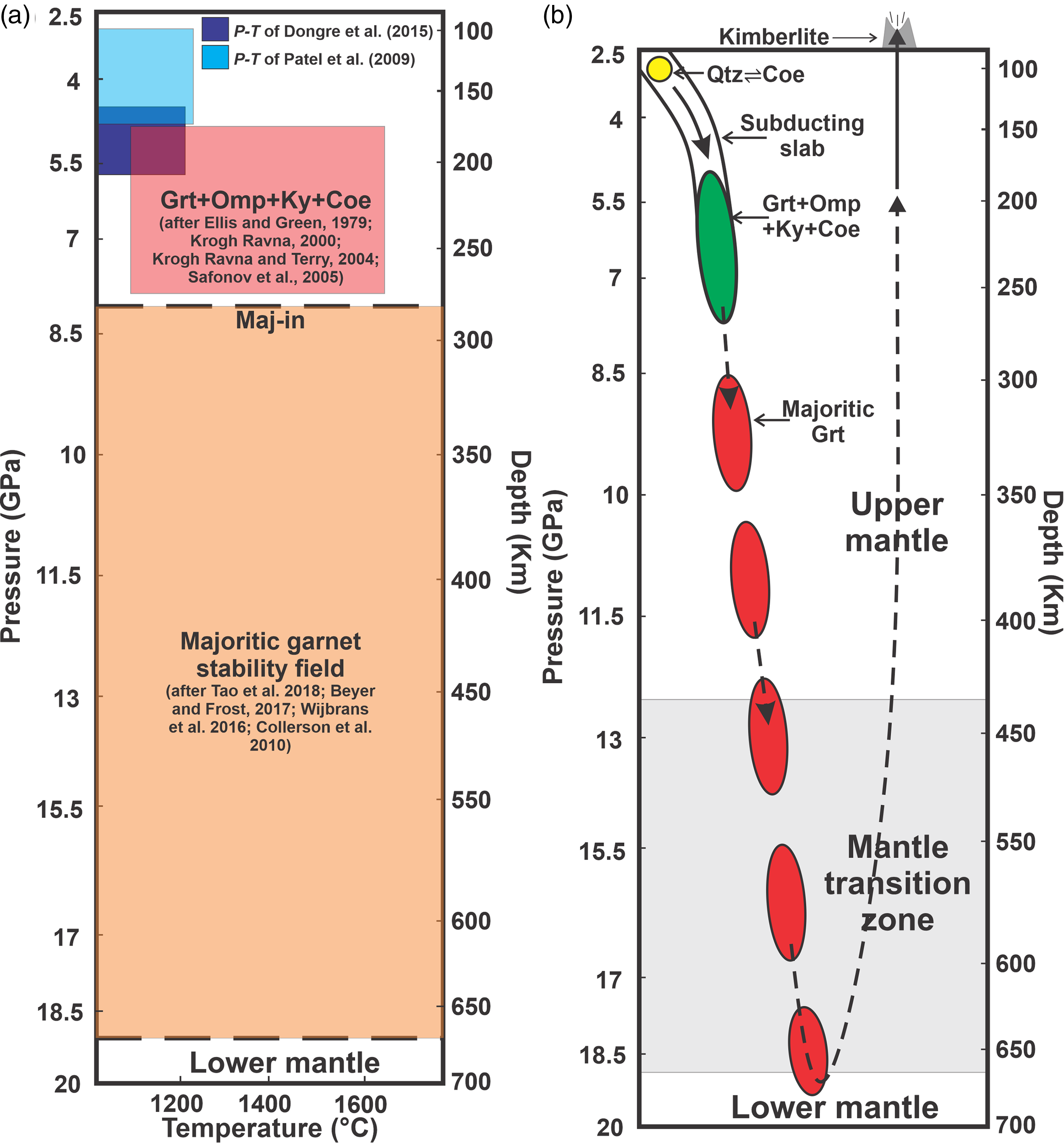
Fig. 11. (a) A pressure–temperature–depth diagram showing the evolution of the subducting crust up to the mantle transition zone. Previous P–T estimation from the eclogite xenoliths of pipe KL-2 is also shown for a comparative understanding. (b) A simplistic diagram showing formation of coesite, K-omphacite and majoritic garnet with increasing depth due to subduction, and a probable transportation path of majoritic garnet from the base of the mantle transition zone (shaded in grey) to the crust through kimberlite eruption.
To constrain the pressure of formation of the majoritic garnets, single-mineral geobarometers based on the Si content of majoritic garnet were used. The formation pressure of majoritic garnet ranges between 9.4 and 17 GPa (after Tao et al. Reference Tao, Fei, Bullock, Xu and Zhang2018), 9.3 and 17 GPa (after Collerson et al. Reference Collerson, Williams, Kamber, Omori, Arai and Ohtani2010), 10.7 and 22.6 GPa (after Wijbrans et al. Reference Wijbrans, Rohrbach and Klemme2016) and 7.7 and 16 GPa (after Beyer & Frost, Reference Beyer and Frost2017).
5. Discussion
The mineralogy of the studied sample is unique for the kimberlite-borne eclogite xenoliths from the Eastern Dharwar Craton as it contains a plethora of UHP minerals such as coesite, K-omphacite and majoritic garnet along with coarse-grained garnet, kyanite and rutile. However, a similar mineralogical assemblage has been reported from the kimberlite-derived eclogite xenoliths from the (i) Kaapvaal craton (e.g. Viljoen, Reference Viljoen1995; Jacob et al. Reference Jacob, Schmickler and Schulze2003; Schmickler et al. Reference Schmickler, Jacob and Foley2004; Shu et al. Reference Shu, Brey, Hoefer, Zhao and Pearson2016), (ii) the West African craton (e.g. Hills & Haggerty, Reference Hills and Haggerty1989) and (iii) the Siberian craton (e.g. Alifirova et al. Reference Alifirova, Pokhilenko and Korsakov2015; Mikhailenko et al. Reference Mikhailenko, Golovin, Korsakov, Aulbach, Gerdes and Ragozin2020). In the following sections, we will discuss the possible implications of the presence of such UHP minerals.
5.a. Implication of coesite
The kimberlite-borne eclogite xenoliths are known to have formed either by subduction of oceanic crust or tectonic emplacement of oceanic crustal cumulates into the mantle (Jacob, Reference Jacob2004; Aulbach & Arndt, Reference Aulbach and Arndt2019). Coesite-bearing eclogites can only form by the subduction of crust at a depth of more than 100 km, and the presence of free silica rules out their origin as high-pressure cumulates (Schulze et al. Reference Schulze, Valley and Spicuzza2000; Jacob, Reference Jacob2004). A high-pressure cumulate origin of mantle eclogites is also not consistent with their geochemistry (see Aulbach & Jacob Reference Aulbach and Jacob2016; Aulbach & Arndt, Reference Aulbach and Arndt2019). The subduction-related origin of the kimberlite-borne eclogite xenoliths from the Kalyandurg cluster is well established based on δ18O in garnet restricted between +5.3 ‰ and +7.8 ‰ (Dongre et al. Reference Dongre, Jacob and Stern2015). A heavy Li isotope signature (δ7Li up to 9.7 ‰) similar to an ancient altered oceanic crust is also observed in the mantle sources of the Wajrakarur kimberlites (Krmíček et al. Reference Krmíček, Magna, Pandey, Chalapathi Rao, Kynický, Krmíček and Chalapathi Rao2022). This study revealed the presence of coesite, which is the first mineralogical evidence in favour of the subduction of oceanic crust of the Dharwar craton, at least, up to ∼100 km.
The presence of monomineralic quartz and bimineralic quartz + coesite (Fig. 6a) along with intragranular fracture (Fig. 4a, b) implies complete to partial transformation of coesite to quartz during depressurization vis-à-vis volume expansion. Since the coesite to quartz transformation is energetically less favourable than the quartz ⇌ coesite, the conversion of coesite to quartz is more common in coesite-bearing ‘orogenic’ eclogites (e.g. Ye et al. Reference Ye, Liou, Cong and Maruyama2001; Perrillat et al. Reference Perrillat, Daniel, Lardeaux and Cardon2003; Gonzalez et al. Reference Gonzalez, Baldwin, Thomas, Nachlas and Fitzgerald2020) owing to their prolonged exhumation history. In contrast, kimberlite-derived coesite-bearing eclogite xenoliths experience rapid ascension from the mantle to the crust (Kelley & Wartho, Reference Kelley and Wartho2000), which helped to preserve the coesite. However, the formation of quartz after coesite is reported from various kimberlite-derived coesite-bearing eclogite xenoliths (Schulze & Helmstaedt, Reference Schulze and Helmstaedt1988; Schmickler et al. Reference Schmickler, Jacob and Foley2004; Mikhailenko et al. Reference Mikhailenko, Aulbach, Korsakov, Golovin, Malygina, Gerdes, Stepanov and Xu2021). Such coesite ⇌ quartz transformation is energetically favourable if the phase transition during depressurization occurs at a temperature >800 °C (Zhong et al. Reference Zhong, Moulas and Tajčmanová2018). The coesite-bearing eclogite xenolith of the present study has been hosted in a kimberlite magma hotter than 800 °C (Kavanagh & Sparks, Reference Kavanagh and Sparks2009) that may have facilitated partial transformation to quartz from coesite. The preservation of coesite is also dependent on the low H2O content (Mosenfelder et al. Reference Mosenfelder, Schertl, Smyth and Liou2005). The absence altogether of hydrous minerals, such as mica and amphibole, suggests the concentration of H2O was low in our sample, which helped to preserve the coesite. The presence of coesite in the eclogite xenolith undisputedly reveals an equilibration deeper than 100 km in the upper mantle.
5.b. Implication of K-omphacite
The pristine cores of the clinopyroxenes of the studied sample revealed that they are potassium-rich omphacites. Potassium-rich clinopyroxenes/omphacites are exclusively found as inclusions in diamond (Safonov et al. Reference Safonov, Bindi and Vinograd2011); however, anomalously higher K2O (3.61 wt %) in natural samples, comparable to those from the present study, are only reported from Kumdy-Kol diamond mine in Kokchetav Complex, Kazakhstan (Bindi et al. Reference Bindi, Safonov, Yapaskurt, Perchuk and Menchetti2003; Fig. 9). In supersilicic clinopyroxenes, the excess silica is accommodated at the M1 octahedral site (Angel et al. Reference Angel, Gasparik, Ross, Finger, Prewitt and Hazen1988; Day & Mulcahy, Reference Day and Mulcahy2007). Experimental and natural system investigations have demonstrated that the formation of supersilicic clinopyroxene requires UHP conditions and temperatures exceeding 1100 °C (Katayama et al. Reference Katayama, Parkinson, Okamoto, Nakajima and Maruyama2000; Okamoto et al. Reference Okamoto, Liou and Ogasawara2000). This is consistent with the absence of phengite in the matrix, which is unstable at temperatures above 950 °C (Okamoto et al. Reference Okamoto, Liou and Ogasawara2000). Although K-enrichment in clinopyroxene is generally attributed to diamond-forming UHP conditions (>5 GPa), it can also be formed in an unusual K-rich environment resulting from metasomatism by potassic melts (Navon et al. Reference Navon, Hutcheon, Rossman and Wasserburg1988; Harlow & Veblen, Reference Harlow and Veblen1991). The absence of K-rich phases such as phlogopite, K-richterite and K–Ba-titanite ubiquitous in potassic melt-induced modal metasomatism (Safonov et al. Reference Safonov, Butvina and Limanov2019) is not consistent with a metasomatism-induced K-enrichment in clinopyroxene in the present case, although the role of cryptic metasomatism cannot be entirely ruled out in the absence of trace-element data.
The P–T estimations involving coarse-grained garnet and K-omphacite along with kyanite and coesite suggest that they were equilibrated at ∼5–8 GPa and ∼1085–1579 °C. Application of the qualitative K-in-pyroxene barometer estimates the pressure between 6 and 7 GPa (Fig. 10). Since K-feldspar in a subducting crust is unstable at >5 GPa, the formation of K-clinopyroxene and coesite at such UHP conditions is explained by the breakdown of K-feldspar into K-clinopyroxene + coesite (Okamoto et al. Reference Okamoto, Liou and Ogasawara2000). Hence, by combining the P–T estimations of different geothermobarometers, we suggest that the garnet–clinopyroxene (K-omphacite)–kyanite–coesite assemblage of our sample equilibrated at ∼5–8 GPa and ∼1085–1579 °C. Compared to the maximum pressure estimations of 5–5.5 GPa by Patel et al. (Reference Patel, Ravi, Thakur, Rao and Subbarao2006, Reference Patel, Ravi, Anil Kumar, Naik, Thakur, Pati and Nayak2009) and Dongre et al. (Reference Dongre, Jacob and Stern2015), this is the highest pressure estimated so far from the xenoliths of the Kalyandurg cluster (Fig. 11a). Our pressure estimation corresponds to a lithospheric thickness of ∼175–280 km and implies that there was a very thick cratonic root with a wide diamond stability window during the kimberlite magmatism at ∼1.1 Ga.
5.c. Implication of majoritic garnet
The studied sample contains fine-grained, Ca-rich majoritic garnet at the periphery of K-omphacite and coarse-grained garnet (Figs 3d, 4c, d). According to the textural relationship between the majoritic garnet, K-omphacite and coarse-grained garnet, it is observed that the majoritic garnet and kelyphitic rim separates K-omphacite from the coarse-grained garnet (Figs 3d, 4c, d), which suggests that the majoritic garnet, along with the kelyphitic rim, formed via reaction between coarse-grained garnet and K-omphacite. The formation of Ca-majorite as a dissociated product of diopside has been already established based on the presence of overlapping peaks between Ca-majorite and diopside (Tomioka & Kimura, Reference Tomioka and Kimura2003; Ghosh et al. Reference Ghosh, Tiwari, Miyahara, Rohrbach, Vollmer, Stagno, Ohtani and Ray2021). The presence of weak anisotropy as observed under the optical microscope (Fig. 4c, d) suggests the absence of cubic symmetry of the majoritic garnet. Such an absence of cubic symmetry is possible either (i) owing to the partial or incomplete transformation from omphacite to majoritic garnet (Xie & Sharp, Reference Xie and Sharp2007) or (ii) by a symmetry reduction from cubic to tetragonal through Mg–Si ordering in the octahedral sites upon cooling (Hatch & Ghose, Reference Hatch and Ghose1989; Tomioka et al. Reference Tomioka, Fujino, Ito, Katsura, Sharp and Kato2002). The textural relationship between the majoritic garnet, coarse-grained garnet and omphacite coupled with the overlapping Raman spectra between the majoritic garnet and omphacite strongly suggests that the studied majoritic garnet formed owing to dissociation of coarse-grained garnet and omphacite with increasing pressure, which also explains the presence of excess Na2O and CaO in such majoritic garnet.
The majoritic garnets are a stable phase at pressures >5 GPa (Van Roermund et al. Reference Van Roermund, Drury, Barnhoorn and De Ronde2000) and are reported from the eclogite xenoliths of other Kalyandurg cluster kimberlites (Pipe P3; Patel et al. Reference Patel, Ravi, Anil Kumar, Naik, Thakur, Pati and Nayak2009). Dissolution of the pyroxene component in the garnet structure with increasing pressure/depth causes four-fold tetrahedral coordination of Si to be converted into six-fold octahedral coordination (Akaogi & Akimoto, Reference Akaogi and Akimoto1977; Irifune, Reference Irifune1987). The presence of Si at the octahedral site in garnet is achieved by (i) the replacement of a trivalent cation (Al3+ and Cr3+) of octahedral coordination by a divalent cation (Mg2+, Ca2+, Fe2+) and Si4+ leading to its supersilicic nature, (ii) Al deficiency and (iii) an increased majoritic component represented by viiiM3vi(Al2-2nMnSin)ivSi3O12, where M is a divalent cation and n ranges between 0 and 1 (Akaogi & Akimoto, Reference Akaogi and Akimoto1977; Moore & Gurney, Reference Moore and Gurney1985; Irifune, Reference Irifune1987). Majoritic garnets are rare in mantle xenoliths, unlike the inclusions in diamonds, but are known from many kimberlite-borne mantle xenoliths (Haggerty & Sautter, Reference Haggerty and Sautter1990; Sautter et al. Reference Sautter, Haggerty and Field1991; Doukhan et al. Reference Doukhan, Sautter and Doukhan1994; Roermund & Drury, Reference Roermund and Drury1998; Xu et al. Reference Xu, Kynicky, Tao, Liu, Zhang, Pohanka, Song and Fei2017). The studied majoritic garnet is rich in CaO unlike the MgO-rich majoritic garnet found in other eclogite xenoliths and diamond inclusions (see Thompson et al. Reference Thompson, Kohn, Prabhu and Walter2021).
A high Na2O in the majoritic garnets points to a high-pressure origin (>6 GPa) corresponding to >200 km depth, well within the diamond stability field (Sobolev, Reference Sobolev1977; Ye et al. Reference Ye, Liou, Cong and Maruyama2001). To constrain the pressure of formation of these majoritic garnets, several single-mineral geobarometers were used. The pressure ranges between (i) 9.4 and 17 GPa (after Tao et al. Reference Tao, Fei, Bullock, Xu and Zhang2018), (ii) 9.3 and 17 GPa (after Collerson et al. Reference Collerson, Williams, Kamber, Omori, Arai and Ohtani2010), (iii) 10.7 and 22.6 GPa (after Wijbrans et al. Reference Wijbrans, Rohrbach and Klemme2016) and (iv) 7.7 and 16 GPa (after Beyer & Frost, Reference Beyer and Frost2017), all of which correspond to a depth range of 280–660 km, up to the base of the upper mantle or mantle transition zone.
The presence of kimberlite-borne eclogite xenoliths confirms the existence of an eclogite reservoir in the lithospheric mantle beneath the Eastern Dharwar Craton, which is a more fertile source for diamond than mantle peridotites (Stachel & Harris, Reference Stachel and Harris2008). Furthermore, a plethora of UHP minerals (coesite, K-omphacite and majoritic garnet) that formed between ∼175 and 660 km preserved in the studied eclogite xenolith point to a viable diamond prospect in the Kalyandurg kimberlites.
5.d. Origin and evolution of the Kalyandurg eclogite
The geothermobarometric estimations obtained from the coarse-grained garnet and omphacite pair, which are in textural equilibrium with kyanite and coesite, revealed that the eclogite formed at ∼5–8 GPa pressure. Thus, the depth of subducted crustal material is estimated as ∼175–280 km. Subsequently, owing to the continued subduction or sinking of the subducted crust after slab break-off, deeper than 280 km, the omphacite and coarse-grained garnet dissociated to form Na–Ca-rich majoritic garnet along the rim of the omphacite and coarse-grained garnet due to increasing pressure. The geobarometric estimations obtained from the majoritic garnet revealed the formation pressure to be 8–19 GPa, which translates to the depth range of ∼280–660 km. Thus, combining the textural observations with geobarometric estimations, it is inferred that the eclogite, once formed owing to ultra-deep subduction between 175 and 280 km, travelled through the mantle transition zone up to ∼660 km, which is manifested by the formation of majoritic garnet from the omphacite and coarse-grained garnet. Hence, this kimberlite-borne eclogite xenolith from the Kalyandurg cluster provides a unique opportunity to investigate the evolution of a subducted crust up to the mantle transition zone.
The studied sample preserves a plethora of UHP minerals that individually bear records of different equilibration depths. For example, quartz to coesite transformation occurred at >100 km (Fig. 11b), whereas the characteristic eclogitic assemblage (garnet–omphacite–kyanite–rutile) formed between 175 and 280 km (Fig. 11b). Continuous increment of pressure after ∼280 km, transformed the omphacite and coarse-grained garnet into majoritic garnet up to ∼660 km (Fig. 11b). Subsequently, after reaching a depth of 660 km up to the mantle transition zone, the eclogite xenolith was brought back to the surface by a kimberlite eruption during Mesoproterozoic time (∼1.1 Ga; Chalapathi Rao et al. Reference Chalapathi Rao, Creaser, Lehmann and Panwar2013) (Fig. 11b). The ascent of eclogite xenoliths from such a great depth to the surface led Paton et al. (Reference Paton, Hergt, Phillips, Woodhead and Shee2007) to suggest that the kimberlite magma of the Kalyandurg cluster originated from the mantle transition zone. However, as the eclogitic lithologies are ductile at sub-lithospheric (convecting mantle) depths, it is difficult to expect such material to undergo fracturing and entrainment in the kimberlitic melt (see Tappe et al. Reference Tappe, Budde, Stracke, Wilson and Kleine2020). It is speculated that the eclogite from the mantle transition zone was carried up rapidly to an intermediate depth (∼200–300 km) by pre-Mesoproterozoic (>1.1 Ga) mantle plume activity that generated widespread mafic dykes in the Dharwar Craton (Samal et al. Reference Samal, Srivastava, Ernst, Söderlund, Srivastava, Ernst and Peng2019). Such a rapid ascent of the mantle plume could possibly prevent the partial melting vis-à-vis melt loss from the eclogite, which in turn, helped to preserve the coesite, K-omphacite and majoritic garnet in the sample. Consequently, the kimberlite magma that originated from such a depth range (∼200–300 km) carried the eclogite as a xenolith (Fig. 11b). The exact process(es) involved during the ascent of eclogite xenoliths from the mantle transition zone to the base of the lithosphere–asthenosphere boundary is difficult to establish and is beyond the scope of this work. Nevertheless, irrespective of the process(es) involved, it is certain that the kimberlite-borne eclogite xenolith evolved near the mantle transition zone as a consequence of pre-Mesoproterozoic ultra-deep subduction before its entrainment in the ∼1.1 Ga old Kalyandurg kimberlite.
Acknowledgements
NVCR thanks DST-SERB, New Delhi for a research project (IR/S4/ESF-18/2011 dated 12.11.2013). NVCR and RP are grateful to BHU for awarding an IoE incentive and Seed grant. AC thanks BHU for a Malaviya Post-Doctoral Fellowship (IoE/MPDF/2020-21/15). Analytical help by Prof. Satyen Saha (Department of Chemistry, BHU) and Dr Koushik Sen (WIHG, Dehradun) is thoroughly acknowledged. Efficient editorial handling by Dr Tim Johnson and critical comments by the reviewer Dr Renée Tamblyn are much appreciated. A previous version of the manuscript was greatly benefited by the comments of Drs Sonja Aulbach, Katie Smart and an anonymous reviewer.