1. Introduction
The advancement of ice sheets and glaciers over unconsolidated sediments may cause coupling between the ice sheet and the underlying sediments. This results in the glacial erosion, deposition and deformation of the sediments and bedrock material (Hart and Boulton, Reference Hart and Boulton1991; Murtaza and others, Reference Murtaza, Dar, Paul, Bhat and Romshoo2021; Dar and others, Reference Dar2022). Widespread structural deformation features in the upper thin lithospheric horizon induced by glacier stress are found within the surficial glaciogenic unconsolidated sediments and adjacent bedrocks in the present and former glaciated areas during the Quaternary Period (van der Wateren, Reference van der Wateren and Menzies1995). Such deformational process as a direct result of prograding glacier movement or loading within the Quaternary sediments and associated bedrocks is recognized as glaciotectonism and acts in the subglacial and proglacial environments (Moran, Reference Moran and Goldthwaite1971; Aber and others, Reference Aber, Croot and Fenton1989; Pedersen, Reference Pedersen1996). Glaciotectonic deformation is a significant process occurring within the glacial environment and has been extensively studied in North America, Europe and the polar regions (Slater, Reference Slater1926; Lundqvist and Lagerbäck, Reference Lundqvist and Lagerbäck1976; Stalker, Reference Stalker1976; Berthelsen, Reference Berthelsen and van der Linden1979; Hart and Boulton, Reference Hart and Boulton1991; Thorson, Reference Thorson2000; Aber and Ber, Reference Aber, Ber, Singh, Singh and Haritashya2011; Vaughan and others, Reference Vaughan-Hirsch, Phillips, Lee and Hart2013; Vaughan-Hirsch and Phillips, Reference Vaughan-Hirsch and Phillips2017; Sigfúsdóttir, Reference Sigfusdottir2019). However, the number of studies that focus on glaciotectonism in the third pole (High-Mountain Asia) in general and the Himalayan and Karakoram ranges in particular remains very limited. Only a few studies on glacier-induced deformations in the regional area have been reported from the Karakoram mountains, Pakistan (Owen, Reference Owen and Croot1988, Reference Owen1989; Owen and Derbyshire, Reference Owen, Derbyshire and Croot1988). Consequently, the identification of glaciotectonic features in Extra Peninsular India in this paper is novel from both a palaeo-glaciological and a palaeoclimatic perspective. The Trans-Himalayan Karakoram Range is associated with some of the largest glaciers outside the polar regions including the Siachen and Baltoro, which have had dramatic impacts on the landscape. Their greater extent in the past and their expansion in the Skardu, Shyok and Nubra valleys of Ladakh are well established (Owen and others, Reference Owen, Caffee, Bovard, Finkel and Sharma2006; Seong and others, Reference Seong2007; Dortch and others, Reference Dortch, Owen and Caffee2010). The confluence zone of the Nubra and Shyok valleys around the Khalsar–Tirith area has been significantly modified by the Quaternary glaciations. The signatures and dimensions of these glaciations have been discussed in several studies (Pant and others, Reference Pant, Phadtare, Chamyal and Juyal2005; Phartiyal and others, Reference Phartiyal, Sharma, Upadhyay, Ram-Awatar and Sinha2005; Owen and others, Reference Owen, Caffee, Bovard, Finkel and Sharma2006; Seong and others, Reference Seong2007; Upadhyay, Reference Upadhyay2009; Dortch and others, Reference Dortch, Owen and Caffee2010; Nagar and others, Reference Nagar, Ganju, Satyawali and Juyal2013). However, the juxtaposition of a large decomposed granite block (8.2 km2) over the Quaternary unconsolidated glaciofluvial sediments on the left bank of Shyok valley between Khalsar village and the T-road junction, puts this landform in a unique perspective. The brittle and ductile deformation features along the contact zone of superimposed decomposed granite and underlying the Quaternary sediments indicate the role of glaciotectonism. Here, based on the extensive field study, we report and discuss the first proposed case of glaciotectonic origin for this landform from the formerly glaciated area within the Trans-Himalayan Karakoram Range, Ladakh, India (Fig. 1). The morphological expressions of glaciotectonic landforms reflecting the subsurface structures are rarely preserved. The surface expression of most of such glaciotectonic landforms is either covered beneath younger deposits or has been significantly modified by the later exogenic processes like glacio-aeolian processes, which are a critical component of glacial and periglacial systems (Derbyshire and Owen, Reference Derbyshire, Owen, Menzies and van der Meer2018). Therefore, the subsurface structure and stratigraphy are invaluable for properly interpreting landforms (Aber and others, Reference Aber, Croot and Fenton1989).
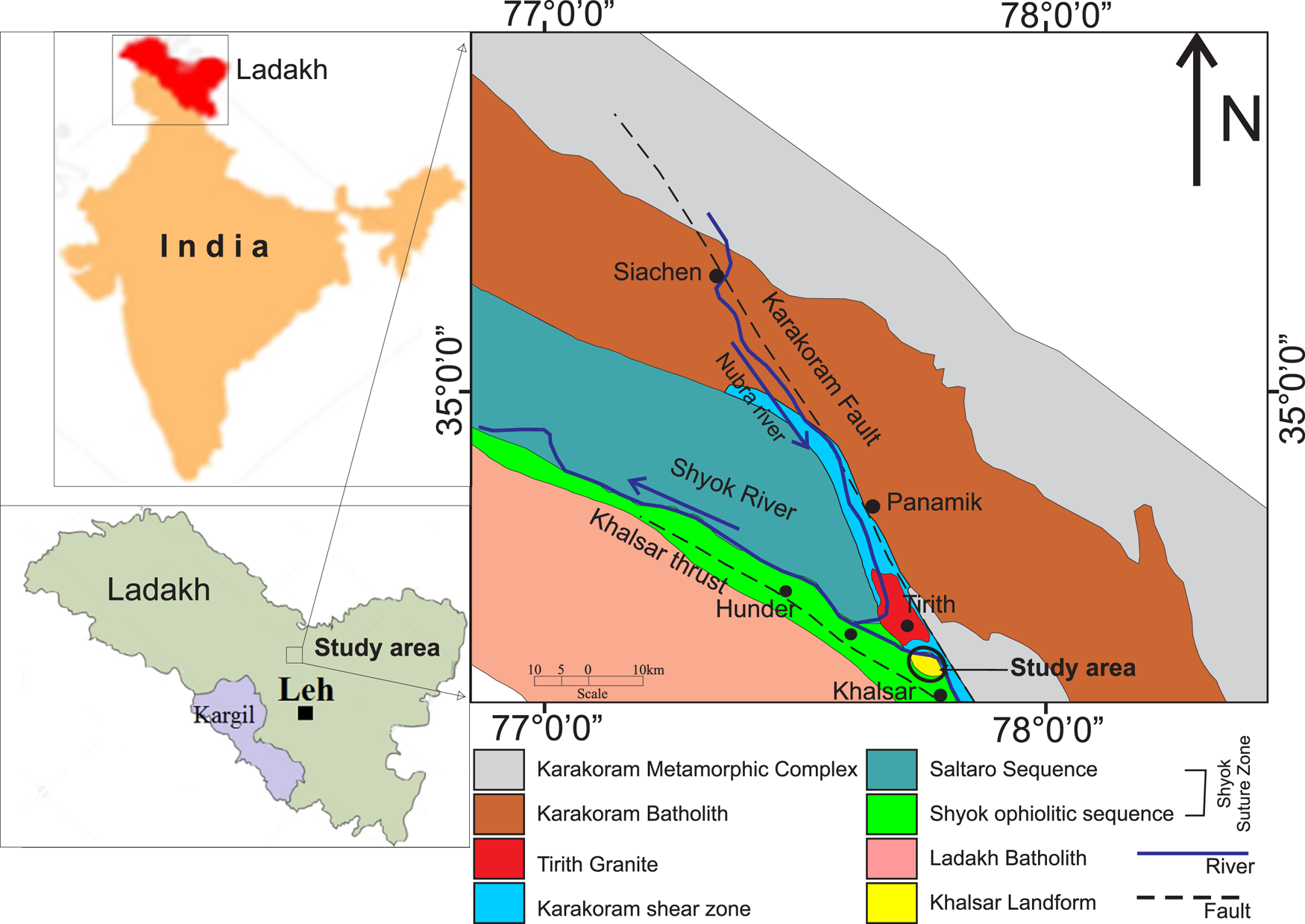
Figure 1. Location and regional geological setup of the study area showing the main lithotectonic units of the Shyok–Nubra high mountain area (modified after Jain, Reference Jain2014; Khanday and others, Reference Khanday, Barman, Dass and Dharwadkar2016).
The identification of glaciotectonic landforms and structures is based on two fundamental criteria: (1) the presence of recognizable masses of pre-existing bedrock and/or drift and (2) the presence of glacially induced deformations within those masses (Aber and Ber, Reference Aber and Ber2007). Such landforms may comprise pre-Quaternary bedrock, pre-existing Quaternary strata usually unconsolidated and/or contemporaneous unconsolidated drift (Aber and others, Reference Aber, Croot and Fenton1989). The glaciotectonic landforms and structures are produced either in situ (autochthonous) within the substratum or detached masses (allochthonous) during their translation and deposition. Distinctive glaciotectonic landforms or ice-shoved hills comprise hill–hole pairs, composite ridges, cupola hills and drumlins. Concealed glaciotectonic structures include megablocks and rafts, diapirs, intrusions and deep basement crustal structures (Clayton and others, Reference Clayton, Moran and Bluemle1980; Aber, Reference Aber and Croot1988; Aber and Ber, Reference Aber, Ber, Singh, Singh and Haritashya2011). Hill–hole pairs represent a basic combination of ice-scooped basin and discrete ice-shoved hill of slightly deformed bedrock or drift material situated next to each other indicating a relatively shorter glacier transport (Bluemle and Clayton, Reference Bluemle and Clayton1984, p. 284). The source depressions are often concealed or modified. Composite ridges or transverse ridges are composite of great slices of up-thrust and commonly contorted sedimentary bedrock that is generally interlayered with and overlain by much glacial drift (Clayton and others, Reference Clayton, Moran and Bluemle1980; Prest, Reference Prest1983). Push moraines are small composite ridges that consists largely or wholly of glaciogenic strata (Prest, Reference Prest1983; Aber and Ber, Reference Aber and Ber2007). The ridges are developed on the crests of folds or the upturned ends of thrust blocks. Folds and thrust blocks that form ridges have usually been detached, transported some distance and stacked up in an imbricated structure. Cupola hills are the irregular jumble of modified ice-shoved hills, having the general characteristics of ice-thrust masses, lacking the hill–hole relationship or the typical ridge morphology (Clayton and others, Reference Clayton, Moran and Bluemle1980; Bluemle and Clayton, Reference Bluemle and Clayton1984). The glaciotectonic origin of such hills can only be proven with evidence of subsurface deformation of bedrock or drift. Glaciotectonic megablocks are large nearly horizontal displaced landforms, slightly deformed and are often buried in glacial strata giving little or no morphologic clue to their presence in the subsurface (Stalker, Reference Stalker1976). Megablocks often form flat-topped buttes, small plateaus or irregular hills, and have been mistaken for in situ bedrock outliers (Jahn, Reference Jahn1950; Stalker, Reference Stalker1976; Sauer, Reference Sauer1978; Ruszczynska-Szenajch, Reference Ruszczynska-Szenajch1987). They are underlain by Quaternary till, gravel or silt. Megablocks and rafts exhibit signs of glaciotectonic deformation in the form of shear zones, folds, faults, thrusting, rotation and brecciation, including fracturing (Aber, Reference Aber1985). Megablocks are generally thin rock slices frozen at the base of the moving ice sheet or glacier, sliding over a décollement within the substratum and could have been transported only by freezing onto the underside of a glacier (Stalker, Reference Stalker1973, Reference Stalker1976). Deposition of detached megablocks occurred when basal melting released it from the ice. Some megablocks may also have been initially pushed in proglacial settings (Ruszczyfiska-Szenajch, Reference Ruszczyfiska-Szenajch1976). Aber (Reference Aber1985) suggested four possible means of megablock entrainment, i.e. beheading of the butte, plucking from lee of the hill, scooping from depression and proglacial thrusting. The initial three processes may occur anywhere under the ice. The transportation distances of megablocks and rafts vary from tens of metres to hundreds of kilometres (Jahn, Reference Jahn1950; Stalker, Reference Stalker1976; Burke and others, Reference Burke, Phillips, Lee and Wilkinson2009). Diapirs, dykes, intrusions and soft sediment deformation features are common within the glaciotectonic landforms and show an injection of one material in a mobile state into the body of another distinct material under dynamic high-glacial stress usually amidst subglacial, water-saturated conditions (Brodzikowski, Reference Brodzikowski1985; Hart, Reference Hart1998).
Glaciotectonic deformations may take place in front of the glacier, beneath the ice margin or under the centre of a thick ice sheet within well-consolidated bedrock to loose Quaternary sediment. Glaciotectonism comprises at least two phases, each characterized by specific diagnostic deformational structures: (1) the proglacial foreland folding and thrusting and (2) the subglacial shearing and cataclastic brecciation (Pedersen, Reference Pedersen1996). Proglacial and subglacial glaciotectonic deformations are induced respectively in the immediate frontal foreland and underlying substratum material by the dynamic forward movement of a glacier generally at shallow levels (Hart, Reference Hart1998). Proglacial glaciotectonic deformations involving longitudinal compression and pure shear are generally characterized by large-scale compressional folds and thrusts and have been observed in many modern glaciers (Gripp, Reference Gripp1929; Gry, Reference Gry1940; Eybergen, Reference Eybergen and van der Meer1987). Subglacial deformation involves simple shear within the underlying substratum and generates folds, shearing, boudinage structures, tectonic banding, laminations and blocks/rafts of chaotically mixed unconsolidated drift sediments and bedrocks (MacClintock and Dreimanis, Reference MacClintock and Dreimanis1964; Lavrushln, Reference Lavrushln1971; Aber, Reference Aber1979; Berthelsen, Reference Berthelsen and van der Linden1979; Boulton, Reference Boulton1979; Rappol and Stoltenberg, Reference Rappol and Stoltenberg1985; Dredge and Grant, Reference Dredge, Grant and van der Meer1987; Hart, Reference Hart1987). Moran (Reference Moran and Goldthwaite1971) suggests that boudinage structures indicating the longitudinal extension deformation could be caused by the shearing movement at the base of the glacier. Glaciotectonic deformation is imposed by the vertical stress due to the static weight of the ice column (glaciostatic pressure) and by the drag or shear stress due to the movement of the ice (glaciodynamic stress) over its bed (Weertman, Reference Weertman1961; van der Wateren, Reference van der Wateren1985). Such deformation produces all sorts of brittle and ductile features of faults, breccia, slickensides, thrusts, dislocations, folds and shear fabrics in thin-skinned unconsolidated sediments, well-indurated strata, to solid crystalline rocks of earth's crust (Aber and Ber, Reference Aber and Ber2007, Reference Aber, Ber, Singh, Singh and Haritashya2011). The base or sole of a glacier is a brittle detachment fault or a slickensided décollement, smeared with fault gouge (Thorson, Reference Thorson2000). A décollement marks the depth of deformation above which strata were displaced and deformed, and below which no deformation took place (Aber and Ber, Reference Aber, Ber, Singh, Singh and Haritashya2011). A décollement generally develops in weak material and can be controlled by the lower boundary of permafrost, lithological contact, the position of confined aquifers or buried hard-rock obstacle (Aber and Ber, Reference Aber, Ber, Singh, Singh and Haritashya2011). Ductile deformation structures mostly occur in unconsolidated or fine-grained strata under high-confining pressures, whereas brittle structures are most characteristic of consolidated or coarse-grained strata, such as sand, and gravel deformed under low-confining pressures (Aber and others, Reference Aber, Croot and Fenton1989). The deformational style between ice-shoved hills and orogenic mountains shows striking similarity (Hopkins, Reference Hopkins1923; Banham, Reference Banham1977; Berthelsen, Reference Berthelsen and van der Linden1979; van der Wateren, Reference van der Wateren1985; Croot, Reference Croot1987; Banham, Reference Banham and Croot1988).
Large-scale glacial erosion and deformation are prevalent under basal sliding and meltwater conditions attributed to the warm basal thermal conditions (Weertman, Reference Weertman1961; Boulton, Reference Boulton, Price and Sugden1972, Reference Boulton and Coates1974; Paterson, Reference Paterson1994). Minimal basal sliding, abrasion and bed deformation are reported beneath cold-based ice conditions due to the minimum glacial movement between ice and the underlying bed (Echelmeyer and Wang, Reference Echelmeyer and Wang1987; Cuffey and others, Reference Cuffey, Conway and Hallet1999, Reference Cuffey2000; Davies and others, Reference Davies, Atkins, van Der Meer, Barrett and Hicock2009). Glaciotectonic deformations may take place under both frozen and thawed conditions during advancing, maximal or recessional phases of glaciations (Sugden, Reference Sugden1977; Aber and others, Reference Aber, Croot and Fenton1989; Waller, Reference Waller2001). The existence of a permafrost layer in front of the advancing glacier above the detachments has been suggested as the cause for the development of large thrust-block moraines by transmitting the stress far into the fore-field of the glacier (Aber and others, Reference Aber, Croot and Fenton1989; Evans and England, Reference Evans and England1991; Boulton and Cuban, Reference Boulton and Cuban1995; Boulton and others, Reference Boulton, van der Meer, Beet, Hart and Ruegg1999; Bennett, Reference Bennett2001). The freezing of sediments and/or bedrock to the base of the glacier has been considered an important prerequisite for the transportation of detached, largely intact glaciotectonic thrust blocks or rafts/megablocks (Clayton and Moran, Reference Clayton, Moran and Coates1974; Banham, Reference Banham, Wright and Moseley1975; Bluemle and Clayton, Reference Bluemle and Clayton1984; Ruszczynska-Szenajch, Reference Ruszczynska-Szenajch1987; Aber, Reference Aber and Croot1988; Burke and others, Reference Burke, Phillips, Lee and Wilkinson2009). However, over-pressurized water within the substratum is suggested to cause the detachment and emplacement of large, unconsolidated thrust blocks over long distances without the ground being frozen (Moran and others, Reference Moran, Clayton, Hooke, Fenton and Andriashek1980; van der Wateren, Reference van der Wateren1985; Broster and Seaman, Reference Broster and Seaman1991; Aber and Ber, Reference Aber and Ber2007; Benediktsson and others, Reference Benediktsson2008; Phillips and Merritt, Reference Phillips and Merritt2008; Benn and Evans, Reference Benn and Evans2010; Vaughan-Hirsch and Phillips, Reference Vaughan-Hirsch and Phillips2017; Sigfúsdóttir, Reference Sigfusdottir2019). High pore-water pressure at the base of glaciers or ice sheets causes decoupling which results in the accelerated glacial flow through basal sliding, enhanced sediment remobilization and deformation because of reduced sediment shear strength along water-lubricated décollements (Piotrowski and Tulaczyk, Reference Piotrowski and Tulaczyk1999; Boulton and others, Reference Boulton, Dobbie and Zatsepin2001; Fischer and Clarke, Reference Fischer and Clarke2001; Kjær and others, Reference Kjær2006; Phillips and others, Reference Phillips, Everest and Reeves2012, Reference Phillips2018; Evans, Reference Evans2018). Such low-friction décollements considerably facilitate the transport of large thrust blocks of sediment and/or bedrock and also affect the style and magnitude of glaciotectonics (Croot, Reference Croot1987; Aber and Ber, Reference Aber and Ber2007; Phillips and Merritt, Reference Phillips and Merritt2008; Burke and others, Reference Burke, Phillips, Lee and Wilkinson2009; Rüther and others, Reference Rüther, Andreassen and Spagnolo2013; Vaughan-Hirsch and others, Reference Vaughan-Hirsch, Phillips, Lee and Hart2013; Vaughan-Hirsch and Phillips, Reference Vaughan-Hirsch and Phillips2017; Sigfúsdóttir and others, Reference Sigfúsdóttir, Benediktsson and Phillips2018).
The main aim of the paper was to report the glaciotectonic activity in the Indian Himalaya, where not much research has been carried out on the subject. The paper reports the field-based sedimentological and glaciotectonic characteristics of the Khalsar landform along Leh–Siachen and Diskit road (NH-1D) near the confluence of Nubra and Shyok valleys and discusses the glaciotectonic source of the landform. The internal glaciotectonic structure of the landform is exposed in the road-cut section on the left bank of Shyok River between Khalsar village and T-road junction and therefore, provides a rare opportunity for its study (Fig. 2). The study, being the first of its kind, will open new research aspects of glaciotectonism in the third pole with wide regional implications.

Figure 2. Google terrain map shows gentle to flat topography of the studied landform and is also supported by the two sections A–B and X–Y across the landform.
2. Regional geological setting
Trans-Himalayan Ladakh lies between the Indian Plate to the south and the Tibetan Plate to the north. To the west, it is separated from the Kohistan arc by the Nanga Parbat syntaxis, and to the east, it is cut off from the Lhasa block by the Karakoram fault (Kowser and others, Reference Kowser, Chandra and Sathyanarayanan2017). The study area lies at the inter-junction of the Karakoram fault, Khalsar fault and Shyok suture zone in the Trans-Himalayan Karakoram Range (Weinberg and others, Reference Weinberg, Dunlap and Whitehouse2000; Yin and Harrison, Reference Yin and Harrison2000; Borneman and others, Reference Borneman2015). The regional area consists of two valleys: (1) the northwest–southeast running Shyok valley drained by the Shyok River, and (2) the north-northwest–south-southeast running Nubra valley drained by the Nubra River. Both valleys exhibit characteristic structural control, primarily associated with the Karakoram fault and the Shyok suture zone, the major regional structural features (Hakhoo and others, Reference Hakhoo2019). The terrain formed by the complex interplay of continental-scale tectonic processes is modified by diverse regional to local glacial, glaciofluvial, lacustrine and aeolian geomorphologic processes (Phartiyal and others, Reference Phartiyal, Sharma, Upadhyay, Ram-Awatar and Sinha2005; Phartiyal and Sharma, Reference Phartiyal and Sharma2009; Dortch and others, Reference Dortch, Owen and Caffee2013; Kumar and others, Reference Kumar2017; Sharma and Phartiyal, Reference Sharma and Phartiyal2018). The area regionally consists of rocks of Ladakh calc-alkaline batholiths towards southwest, the Karakoram metamorphic complex (dominantly metasedimentary and later Karakoram batholiths) towards east and northeast and the ophiolite sequence of melange, basalt including Khardung basalt, gabbro, pelagic to continental shelf sedimentary rocks of Shyok suture zone along with Saltoro molasse towards northwest (Fig. 1) (Robertson and Collins, Reference Robertson and Collins2002; Searle and others, Reference Searle2010; Horton and Leech, Reference Horton and Leech2013; Upadhyay, Reference Upadhyay2014; Borneman and others, Reference Borneman2015; Khanday and others, Reference Khanday, Barman, Dass and Dharwadkar2016). Also, the rocks of Tirith granite nearby have been differently correlated with Ladakh and Karakoram batholiths (Jain, Reference Jain2014; Khanday and others, Reference Khanday, Barman, Dass and Dharwadkar2016). Further, Pleistocene and Holocene deposits of an unconsolidated moraine, till, lacustrine, glaciofluvial and aeolian deposits are also present (Phartiyal and Sharma, Reference Phartiyal and Sharma2009; Dortch and others, Reference Dortch, Owen and Caffee2010; Scherler and others, Reference Scherler2014; Ganju and others, Reference Ganju, Nagar, Sharma, Sharma and Juyal2018). Pant and others (Reference Pant, Phadtare, Chamyal and Juyal2005) and Dortch and others (Reference Dortch, Owen and Caffee2010) interpreted the Khalsar deposit as lateral moraine. However, Phartiyal and others (Reference Phartiyal, Sharma, Upadhyay, Ram-Awatar and Sinha2005) and Scherler and others (Reference Scherler2014) interpreted it as a landslide deposit. Based on the presence of lacustrine sediments along the Shyok valley, a palaeolake formation near Khalsar village has been suggested by the damming of the Shyok River due to tectonic activity or blocking by Siachen Glacier (Phartiyal and others, Reference Phartiyal, Sharma, Upadhyay, Ram-Awatar and Sinha2005; Dortch and others, Reference Dortch, Owen and Caffee2010).
3. Methodology
The present study is based on extensive fieldwork to identify geomorphological features, lithological features, structural fabric, glaciotectonic features and their interrelation from the Khalsar landform within the Trans-Himalayan Karakoram Range. The landform shows lithologies of unconsolidated Quaternary sediments and decomposed granite megablocks. The sedimentological characteristics of the unconsolidated Quaternary deposits at the Khalsar landform were described using the lithofacies coding and terminology of Miall (Reference Miall1977) and Eyles and others (Reference Eyles, Eyles and Miall1983). The gravel has been used for the moderately sorted sub-rounded imbricated fluvial unit. The term diamicton (or diamict) has been used as the non-genetic term for the poorly sorted clast–sand–mud admixture (Eyles and others, Reference Eyles, Eyles and Miall1983). The gravel bed is horizontal represented as Gm, whereas the sand bed showing horizontal laminations is represented as Sh. Diamicton shows varieties of matrixes supported by highly unsorted massive diamicton (Dmm), matrix-supported graded slightly stratified (Dmg/Dms) and matrix-supported sheared, faulted with slickensides (Dmm(s)).
The lithologies and deformational features observed during the field study are shown in the lithological logs and photomontage. The lithological log was prepared by measuring the true thickness of horizontally disposed lithological units in the vertical section, and after recording their sedimentological and deformational characters. The nomenclature used by Miall (Reference Miall1977) and Eyles and others (Reference Eyles, Eyles and Miall1983) was used for describing the individual lithological units. The photomontage was prepared by coalescing the various field evidence viz. sedimentological, structural and lithological, to understand the role of glaciotectonism in the formation of the landform. The lithological log and the sectors exposing the deformational features of the fault zone with slickensides, glaciotectonite (cataclastic breccia), mylonitic fabric at contact with granite rafts and diamicton, shear bands and thrust features were demarcated in the photomontage (Boulton and others, Reference Boulton, Dent and Morris1974; Bluemle and Clayton, Reference Bluemle and Clayton1984; Croot, Reference Croot1987, Reference Croot and Croot1988; Aber, Reference Aber and Croot1988; Banham, Reference Banham and Croot1988; Hart and Boulton, Reference Hart and Boulton1991; Phillips and Auton, Reference Phillips and Auton2000; Burke and others, Reference Burke, Phillips, Lee and Wilkinson2009; Pedersen, Reference Pedersen2014). The direction of shear sense in shear bands, ductile fold noses, clastic dykes and tectonically imbricated clasts of diamicton was recorded and shown on field photos. The probable source and direction for the shear stress were deciphered. The critical analysis of lithological, sedimentological and structural data and their synthesis in the form of lithological logs and photomontage led to the development of a preliminary model for the glaciotectonic origin of the Khalsar landform.
4. Results
During the present study, the surface geomorphological and topographical characteristics were analysed for the entire landform. However, the detailed sedimentological and structural studies were carried out only in the northern half section of the landform towards T-road junction. The northern half section of the landform forms a vertical road cut section and exposes various lithologies and structural features in the fresh cross-sectional view of the landform, thereby making the landform suitable for sedimentological and structural studies. The thick recent scree and alluvium material covering the southern half section towards Khalsar village restricts it from detail study. Therefore, only the northern half section of the landform has been covered during the present study.
4.1. Geomorphological characteristics
The Khalsar landform falls in an arid cold desert barren terrain between elevation ranges of 3100 and 3600 m above mean sea level, where maximum and minimum temperatures for the last 40 years varied between −10 to 15°C and −35 to −5°C (https://power.larc.nasa.gov/data-access-viewer/). The landform has an 8.2 km2 area with a maximum length of 4 km and has an average thickness of 200 m in the road cut section, and its thickness decreases to <20 m against the basalt hills on the western side (Fig. 2). The surface topography of the landform varies from slightly uneven to flat, with rolling topography at places (Figs 2, 3). The surface of the Khalsar landform shows frost shattered and weathered low-amplitude hummocky granite exposures, with widespread disintegrated boulders (Figs 3a, b). The individual hummocky bedforms are quasi-circular, with a few slightly elongated in the west-northwest direction, and are suspected to be the remnants of roche moutonnées (Figs 3a, b). The surface morphology of these disintegrated granite mounds is extensively modified by glacio-aeolian processes. The original bedrock exposures with smooth glacial surfaces are roughened by exogenic processes and are fragmented by the frost action (Figs 3c–e). The aeolian activity is shown by the features of deflation hollows and desert pavement in the disintegrated granite blocks (Figs 3e, f). The prevalence of aeolian activity and frost action reveals the cumulative role of glacio-aeolian processes in shaping the present topography of the Khalsar landform.
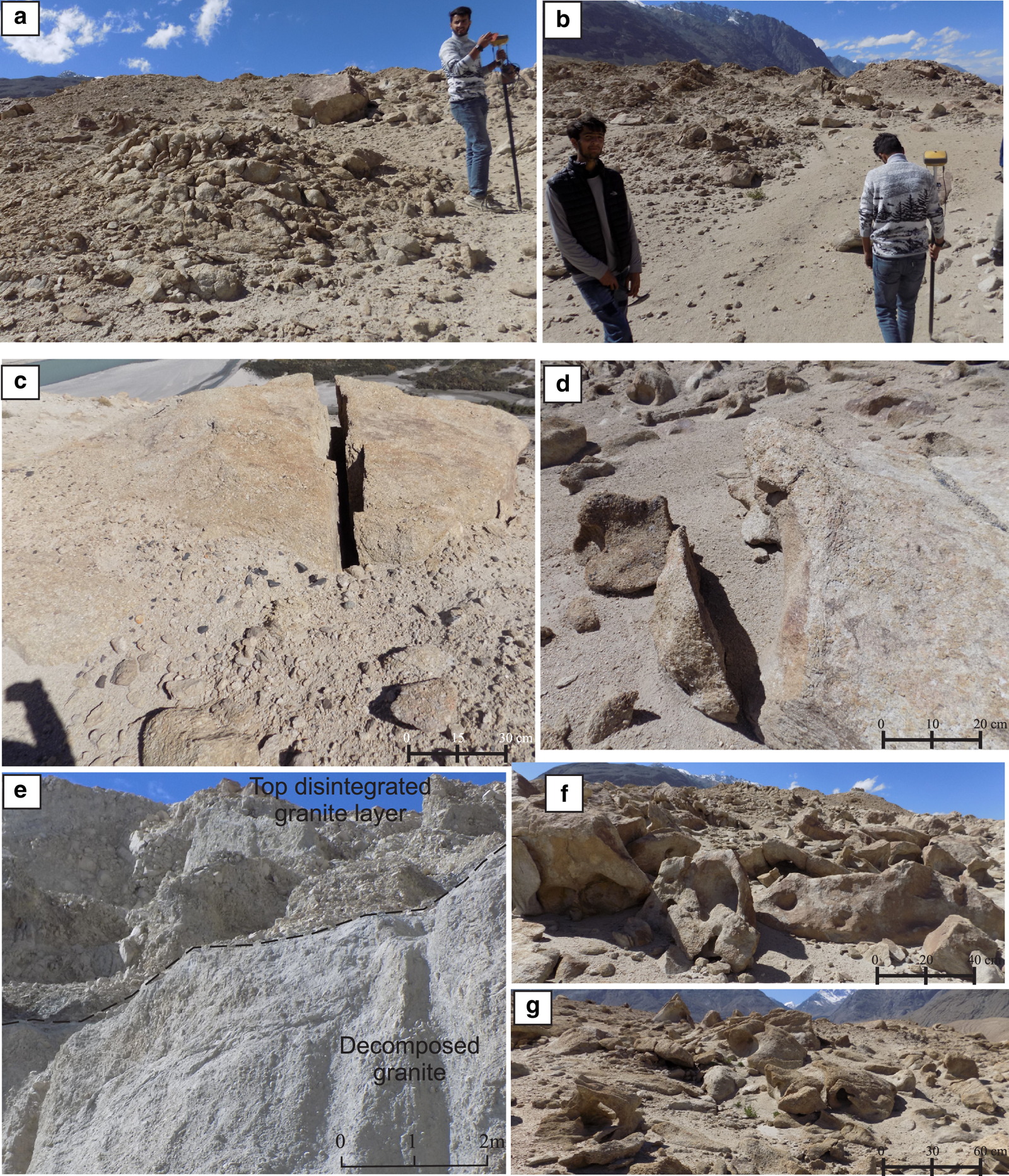
Figure 3. Field photographs showing various morphological features of the Khalsar landform. (a, b) Small elongated hummocks of highly disintegrated granite suspected as remnant roche moutonnées, (c, d) remnant granite blocks disintegrated by freeze–thaw action, (e) granite megablock shows contact of highly decomposed and grussified disintegrated layers respectively in bottom and top and (f, g) deflation hollows by aeolian activity in decomposed and disintegrated granite.
4.2. Lithological observations
The Khalsar landform, from bottom to top comprises unconsolidated Quaternary sediments including undisturbed fluvial sediments of the boulder/gravel (Gm) and sand (Sh) beds, highly disturbed diamicton deposits (Dmm) and rafts of granite and slate, very large decomposed granite (DGR) body along with diamicton (Figs 4–8). The unconsolidated Quaternary deposits show fluvial and glacial environmental signatures (Figs 5–7). The granite body overlies the unconsolidated fluvial and diamicton sediments towards the northern cliff side along the road section and on Khardung basalt along the Khalsar Nala behind the Khalsar village (Fig. 4a). The thickness of the landform decreases southwards against the basaltic hill. Isolated bodies of debris-rich material occur within the granite and diamicton.
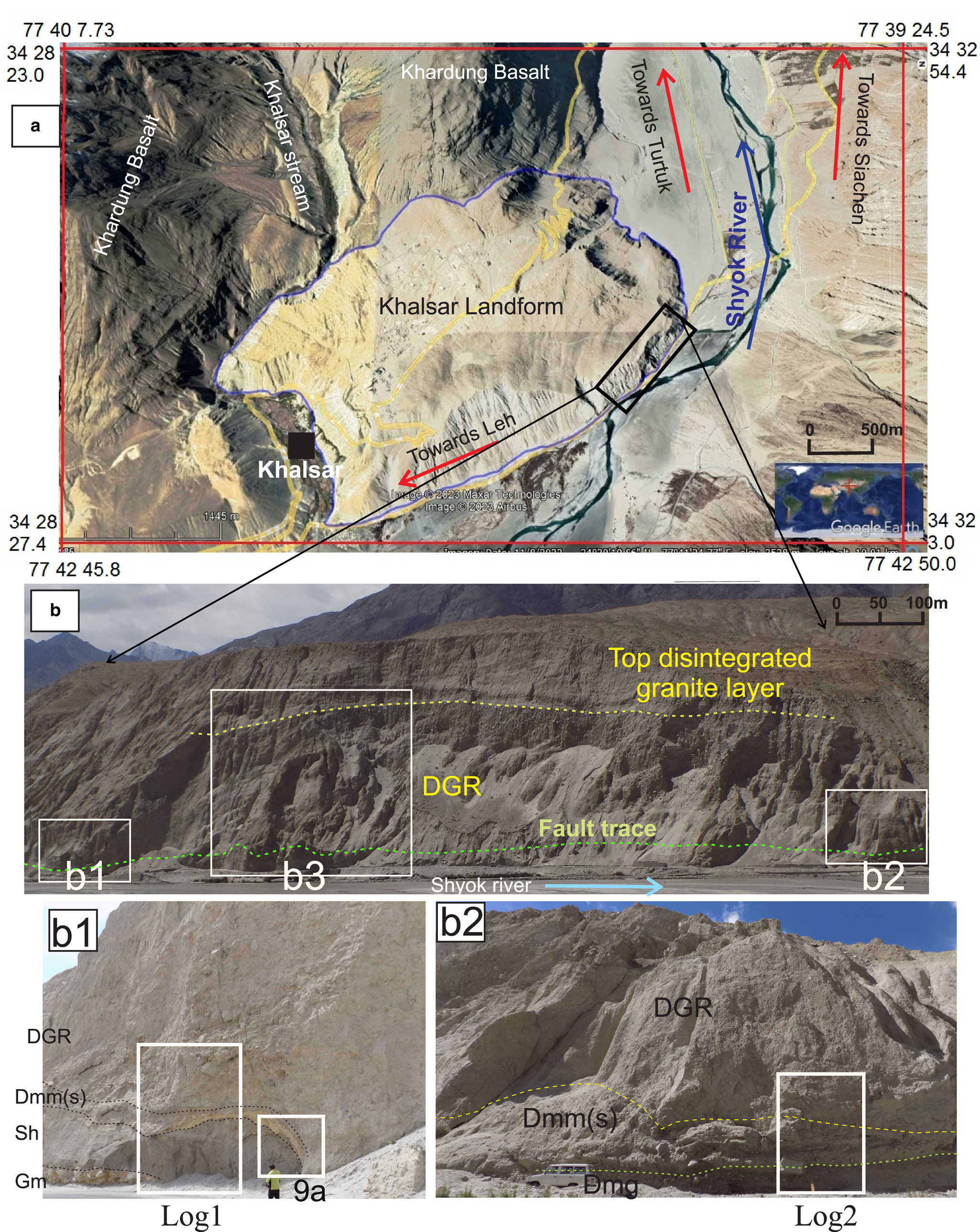
Figure 4. (a) Google Earth view of the study area (March 2023) showing the Khalsar landform. To its south Khardung Basalt of Shyok suture zone is exposed, (b) field photos of Khalsar landform show a vertical slope (cliff), with a nearly flat to very gentle top and is covered by dry ravels at slope breaks. The dashed lines separate the lowermost diamicton unit from the overlying decomposed granite by fault. The top disintegrated and grussified granite layer is also separated from the decomposed unit. (b1), (b2) and (b3) mark the sections studied. (b1) Bottom to top: Fluvial sediments of gravel bed (Gm), sand bed (Sh), massive unsorted brecciated diamicton (Dmm(s)) and decomposed granite. (b2) Bottom to top: Graded and unsorted brecciated diamicton (Dmg/Dmm(s)) and decomposed granite. (b3) Refer to Figures 8, 10 and 11.

Figure 5. Lithological log-1 (refer to Fig. 4b1) showing the disposition of the various lithological units of the Khalsar landform which include Quaternary fluvial sediments (Gm/Sh), diamicton (Dmm) and granite (Gr) along with field photographs from bottom to top.

Figure 6. Lithological log-2 (refer to Fig. 4b2) showing the disposition of the various lithological units of the Khalsar landform which include Quaternary diamicton (Dmm/Dmm(s)/Dmg/Dms) and granite (Gr) along with field photographs from bottom to top.

Figure 7. Field photos showing (a) undisturbed fluvial sediments of boulder (Gm) and sand (Sh) beds overlain by diamicton (Dmm(s)) along with fault gouge (FG) and decomposed granite (DGR), (b) large slate raft (Rft) within the diamicton (Dmm) with fault gouge at the base of raft and (c) decomposed granite over the disturbed diamicton (Dmm(s)) with a non-planar contact in between.

Figure 8. Field photos showing characters of Grussified, decomposed and disintegrated granite. (b3) Photomontage of decomposed and disintegrated granite at Khalsar cliff showing various glaciotectonic field features, (a) the cliff section exposes the three characteristic layers of granite exposure, L1, L2 and L3 with the bottom layer showing more decomposed character (DGR), (b) development of rills in the vertical slope of decomposed granitic indicates its highly altered nature, the granitic characters of massive to jointed nature are lacking and (c, d) large granite rafts (Rft) within the highly unsorted clay dominated diamicton (Dmm).
4.2.1. Fluvial sediments
The fluvial sediments include the gravel or bolder bed (Gm) and sand bed (Sh). The gravel or boulder bed (Gm) marks the bottommost unit containing polymictic sub-rounded matrix-supported boulders (up to 60 cm), cobbles and pebbles, and has a sharp horizontal contact with the overlying sand bed. The boulder bed shows a yellow to rusty colour. This bed is horizontal and moderately sorted with crude imbrications of clasts (Gm). The sand bed (Sh) is thick, loose massive and horizontally laminated with occasional pebbles. Sand shows a slightly graded nature from gritty sand at the bottom to coarse sand in the middle. The gritty nature reappears towards the top also. The contact of the sand bed (Sh) with the overlying diamicton bed (Dmm) is sharp curvilinear undulatory to faulted in nature (Figs 5, 7a).
4.2.2. Diamicton bed (Dmm)
The diamicton bed (Dmm) is a matrix-dominated unit showing angular, sub-angular to sub-rounded gravel, pebbles and large boulder-sized clasts (30–50 cm) disseminated within the unsorted fine sand, silt to clay dominated matrix (Figs 5–7). Substantial clay to silty clay fraction in the matrix is present at some exposures. The diamicton bed shows the unconsolidated massive and highly unsorted character (Dmm). At places, diamicton exhibits moderate graded to slightly stratified character with occasional sandy lenses and stringers (Dmg/Dms). A fault zone (FG) with slickensides has developed within the diamicton above which it is deformed and disturbed (Dmm(s)). The diamicton sediments below the fault gouge are undisturbed and preserve the grading and stratification (Dmg/Dms). However, a large diamicton boulder has distorted the primary fabric of the underlying unit due to its load on soft sediments rather than structural deformation (Figs 6, 7c). Small-to-large boulder-sized rafts of granite and slate are also scattered within the diamicton unit (Figs 7b, 8c, d). The gravel-rich diamicton shows imbrication along the ductile deformation zones (Fig. 10). The clay matrix around a few large boulders of diamicton shows sigmoidal swerving of ductile shear fabric. The contact of diamicton with overlying granite is sharp curvilinear sheared to faulted.
4.2.3. Granite
The granite (DGR) exposure overlying the diamicton unit (Dmm) has an average thickness of 180–190 m in the eastern cliff section which decreases westwards against the underlying basalt. The granite body is not connected to the root zone and overlies the Quaternary unconsolidated sediments (Figs 4b, 5, 6). Based on the mechanical strength and physical appearance, the granite body is divided into three parts: (1) a mechanically disintegrated, thin blocky granite layer at the top, (2) a widespread decomposed granite layer at the bottom and (3) a very small highly jointed compact granite exposure towards the eastern side near the Khalsar village having moderate strength (Figs 3e, 8a, b). A megascopic study of compact granite reveals the presence of quartz, k-feldspar, plagioclase feldspar and minor muscovite. It also displays the characteristic features of granite, like strength, consistent sharp-edged to sub-rounded jointing and magmatic textures. The top layer in the plane shows enclaves of mica schist within highly disintegrated granite. The bottom layer of decomposed granite is the widest spread unit and is significantly altered. The frost action and grussification process are the prima facie for the highly disintegrated nature of the decomposed granite up to a much deeper (>200 m) level (Figs 3c–e, 4b). The term ‘gruss’ refers to the fragmental product of the granular disintegration of granitic rocks in particular and other rocks in general (Bates and Jackson, Reference Bates and Jackson1987; Kajdas and others, Reference Kajdas, Michalik and Migon2017). The granitic gruss origin has been attributed to the physical weathering through insolation or frost action by various researchers (Johannson, Reference Johansson1934; Marchand, Reference Marchand1974). The granular disintegration of granite exposures in the Khalsar landform has resulted in the accumulation of extensive talus at foot slopes and slope breaks (Figs 4b, 8a). It also exhibits the hard blocks (core stones) surrounded by the matrix of gruss (granite decomposed to dense sandy soil). The decomposed granite due to grussification has lost the physical and mechanical characteristics of the sheet, exfoliation and polygonal jointed character, high strength and compactness (Figs 4b, 8a, b). Most of the joint traces are lost due to the extensive decomposition with a few poorly preserved and discontinuous. The magmatic texture on the surface is altered and is visible only after the fresh surface is exposed. The decomposed granite easily gets scratched with a hammer and can be excavated by JCB machines/excavators. However, it, although weak, still withstands the vertical cut slope, which is attributed to the cohesion of the feldspar alteration products. The steep slopes of decomposed granite show the development of rill and gully erosion, which is very uncommon in compact hard granite and is, therefore, attributed to its decomposition, grussification and feldspar alteration (Figs 4a, b, 8b). A thin intermediate layer, existing between the upper disintegrated and lower decomposed granite layers, shows highly angular fragments of granite (Fig. 8a). The inaccessible layer visible in the cliff section may have been an active layer under permafrost conditions as indicated by the mechanical weathering characteristic of frost action.
4.3. Structural features
The megascopic deformational features found in the Khalsar landform include brittle fault features, ductile shear fabric and soft-sediment deformation (SSD) structures at the contact between the underlying diamicton (Dmm) and overlying decomposed granite (Figs 9–11). At places, the contact of diamicton (Dmm) with decomposed granite (DGR) is highly non-linear undulatory and shows thrust-related ductile deformation features (Figs 9d, e, 10). Further, variable dimensional rafts of granite (Rft) and slate (Rft(Sl)) have been found within the diamicton (Figs 7b, 8c, d, 10c). The superimposition of the granite megablock (DGR) over the underlying multiple lithological units of Quaternary fluvial sediments, diamicton deposits and basalt indicates its allochthonous origin during the Quaternary time.
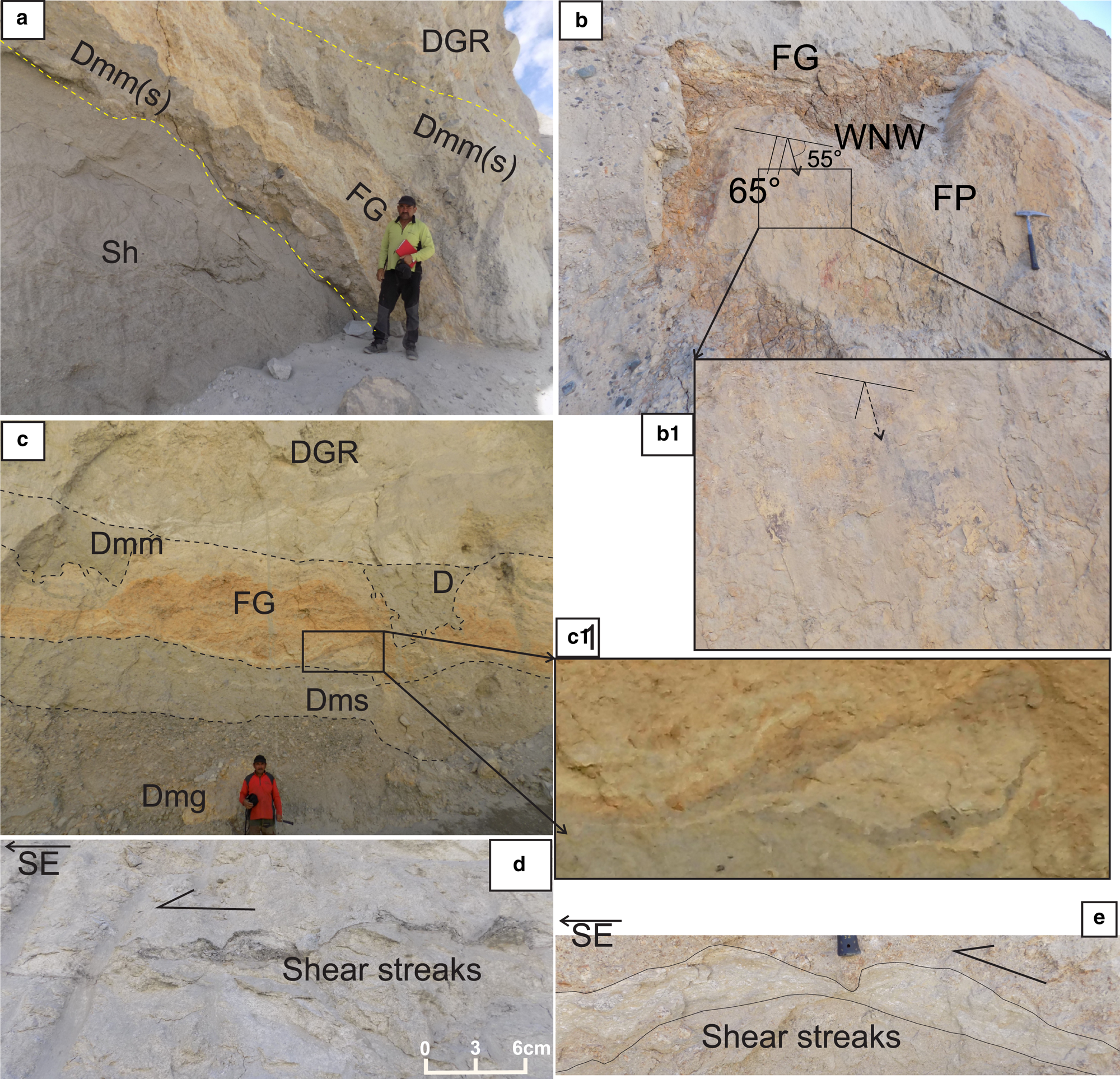
Figure 9. Field photos showing brittle and ductile deformation features in Khalsar landform. (a) Decomposed granite (DGR) along with diamicton (Dmm(s)) thrust over the undisturbed sand bed along a fault zone represented by fault gouge within the diamicton (FG), (b) the fault plane, although poorly exposed shows slickensides, (c) decomposed granite (DGR) shows sharp faulted contact with underlying graded and stratified diamicton (Dmg/Dms), which is also injected within fault gouge (inset), (d) thin shear seam within the decomposed granite shows near the flat base and folded top indicating thrust propagation fold nature, the attenuation of amplitude in folds towards southeast indicate top to southeast movement and (e) the shear seam shows attenuation of amplitude in middle portion due to the deflection by competent remnant granite block within the decomposed granite, the drag in upper contact indicates top to southeast movement.

Figure 10. Field photos (refer to b3 in Fig. 4b) showing ductile glaciotectonite deformation features in Khalsar landform. (a) Unconsolidated diamicton (Dmm(s)) dragged and narrowing up within the decomposed granite (DGR) towards southeast, the sheared portion (SDG) and drag shows top to southeast movement direction, the presence of large boulders and apophyses of diamicton within the decomposed granite indicates sufficient stress and saturated nature of the material, boudinaged and stretched granite material within shear material indicates ductile behaviour of the material attributed to the glacial stress and partially frozen material, (b) narrowing up diamicton dyke and (c) large boulders of slate and quartzite imbricated within the southeastward dragged diamicton material, the cartoon (after Hart and Boulton, Reference Hart and Boulton1991) for thrust propagated leading edge fold model indicates top to southeast thrust direction.

Figure 11. Field photos (refer to b3 in Fig. 4b) showing ductile glaciotectonite and SSD features in Khalsar landform. (a–c) Thin cataclasite glaciotectonite zone at the contact of diamicton (Dmm(s)) and decomposed granite (DGR), the cataclasite shows mylonite like ductile fabric with feldspar porphyroclasts within the granite and mud dominant matrix, indicating sufficient strength in the diamicton material to undergo ductile deformation at the surficial level, which is attributed to its frozen to partially frozen nature, (d–f) the diamicton water escape structures and dykes with large boulders indicate partially frozen slurry material under very high stress.
4.3.1. Brittle deformation
A cataclastic zone, with a conspicuous fault gouge (FG), exists between the underlying Quaternary fluvial sediments (Gm/Sh) ± diamicton sediments (Dmm), and overlying granite body (DGR) ± diamicton (Figs 5, 6, 7a, b, 9a, c). It shows a well-preserved fault gouge and breccias of 1–1.5 ft thick and rare slickensides along the poorly preserved fault plane. The fault gouge shows broken and crushed fragments of granite and diamicton within the finer ground and pulverized material. The lower part shows a fault brecciated character with more broken fragmented material. The granulation and powder character with yellow colour in the cataclastic zone is observed more towards its upper contact with the granite. The fault plane shows a strike of west-northwest–east-southeast dipping 45–75° towards the north (Fig. 9b). The pitch of the slickensides is 55° towards 280°. Further, the chatter marks indicate glacier movement towards the south, opposite to the dip direction of the fault plane. The overriding of Quaternary sediments (Gm/Sh/Dmm) by older granite (DGR), and movement direction opposite to the dip direction of the fault plane indicates reverse to thrust faulted origin of the fault gouge zone (FG).
4.3.2. Ductile deformation
Two well-developed megascopic shear fabric patterns have been identified in the exposure, both showing the top to the south direction. Thin shear bands and zones are consistently found within the decomposed granite (Figs 9d, e). The thicker shear zones (SDG) show a nearly linear flat base and curved convex top with south vergence, indicating it is a glaciotectonic fault-related fold (Figs 9c, d, 10a2). The contact between the diamicton sediments and decomposed granite shows asymmetrical antiformal drag folds, ductile shearing, boudinaged shear lenses and thrusting. The diamicton sediments (Dmm) show conspicuous convex up drag fold noses protruding within the overlying decomposed granite (DGR). Such features within the overlying decomposed granite consistently show southward dragging and tilting. These features have been related to the prolonged thin skin glaciotectonic thrust propagation folds (Figs 10a, c). The thrusting and shearing have led to the dragging and alignment of pebbles and boulders of diamicton (Dmm(s)) within the overlying decomposed granite (Figs 10a, c). The dragged contacts between unconsolidated Quaternary diamicton (Dmm(s)) and decomposed granite (DGR) also show the shear fabric of sigmoidal and delta-type porphyroclasts of feldspar within the high-strain zone of mixed granitic and clay groundmass (Figs 11a–c). The presence of such a narrow mylonite fabric indicates sufficient competence in the rheologically ductile clay-dominant diamicton, which is attributed to its subglacial or proglacial partial frozen condition. The overall appearance and deformation intensity in small-scale ductile fabric present in the Khalsar landform is similar to the chalk hill of northeast Norfolk, UK (Burke and others, Reference Burke, Phillips, Lee and Wilkinson2009). The presence of diamicton (Dmm) all along the fault, shear and thrust zones, verging shear fabric in fault-related folds, verging thrust propagation drag folds and ductile-sheared porphyroclast indicates the role of thin skin glaciotectonic thrusting in the emplacement of the Khalsar landform rather than orogenic thrusting.
4.3.3. Soft-sediment deformation (SSD) structures
Few thick (20–30 cm) clastic pebbly diamicton dykes (DD) and water escape structures, attributed to the hydrofracturing, are found cutting across the decomposed granite (Figs 10b, 11d–f). Some diamicton dykes and fingers within the decomposed granite show south verging. Further, certain decomposed granite and Quaternary sediment exposures also show suspected permafrost irregular involution and cryoturbation features. The involutions are related to the cryogenic active layer in permafrost, with deeper involutions attributed to soft sediment deformation during later permafrost degradation (Svensson, Reference Svensson1990; Goździk, Reference Gozdzik1995; Murton, Reference Murton2001). The intermediate layer between the disintegrated and decomposed granites is also suspected as a permafrost active layer at the thaw boundary where the active and frozen layers meet.
5. Discussion
5.1. Glaciotectonic origin
The landform located between the Khalsar village and the T-road junction near Shyok and Nubra river confluence (Figs 1, 2) shows the juxtaposition of the hard rock of decomposed granite over the Quaternary unconsolidated diamicton (Dmm/Dmm(s)) and/or fluvial sediments (Gm/Sh). The presence of ductile and brittle deformation features of mylonite and basal brittle fault gouge (FG) within the contact zone of unconsolidated diamicton and overlying decomposed granite has put this Quaternary landform in an unusual perspective (Figs 4–11). The occurrence of thin high-strain structurally deformed strata above the fault gouge zone indicates the role of the thin skin thrusting phenomenon. However, the thin skin deformation in the ultrathin peel of diamicton and decomposed granite (avg. 200 m) above the fault gouge and no such deformation in underlying fluvial sediments and diamicton is very unusual and may not be related to orogenic tectonic activity. The dispersal of diamicton over the fluvial sediments and under the decomposed granite indicates the prevalence of formerly glaciated and/or periglacial conditions and, therefore, signals the role of glaciotectonism in such thin skin deformation. Pertinently, glaciotectonics involves glacially induced deformations in the earth's crusts, which include all manners of folds, faults, contortions, intrusions, diapirs and dislocations and are typical of unconsolidated or fine-grained strata that are deformed under high-confining pressures (Aber and Ber, Reference Aber and Ber2007, Reference Aber, Ber, Singh, Singh and Haritashya2011). Widespread shallow glaciotectonic ductile and brittle deformation features (folding and thrusting) due to the glacier loading and movement on the earth's thin crustal surface are reported from the former and modern glaciated areas (Hart and Boulton, Reference Hart and Boulton1991; Waller and others, Reference Waller, Murton and Whiteman2009; Aber and Ber, Reference Aber, Ber, Singh, Singh and Haritashya2011; Vaughan-Hirsch and Phillips, Reference Vaughan-Hirsch and Phillips2017). The thin-skinned thrust tectonic model for understanding the glaciotectonic deformation is, therefore, comparable to foreland fold-and-thrust belts developed in response to crustal shortening and mountain building on a much smaller and shallower scale (Berthelsen, Reference Berthelsen and van der Linden1979; Croot, Reference Croot1987; Pedersen, Reference Pedersen, Jones and Preston1987, Reference Pedersen, Goldthwait and Matsch1988, Reference Pedersen2000; van Gijssel, Reference van Gijssel and van der Meer1987; Banham, Reference Banham and Croot1988; Aber and others, Reference Aber, Croot and Fenton1989; Bennett, Reference Bennett2001; Andersen and others, Reference Andersen, Hansen and Huuse2005; Vaughan-Hirsch and Phillips, Reference Vaughan-Hirsch and Phillips2017). The glaciotectonic structures also include megablocks and rafts, diapirs and wedges as well as SSD structures (Aber and Ber, Reference Aber, Ber, Singh, Singh and Haritashya2011). The Khalsar landform, containing the coherent mass of granitic bedrock along with diamicton sediments, is well traversed by ductile shear zones, faults and brecciated zones, and has thrust propagated drag fold noses, conspicuous megablocks and rafts formed under the subglacial setup (Evans, Reference Evans, Elias and Mock2013). The area also shows the preservation of graded and stratified diamicton units (Dmg/Dms) and undisturbed sand and gravel beds (Sh/Gm) below the fault gouge. The clastic dykes, water escape structures and narrow linear fingers (Figs 10b, 11d–f) indicate hydrofracturing of granite during glaciotectonism by pressurized meltwater injection into the rocks at the base of glaciers as reported by several researchers elsewhere (Boulton, Reference Boulton and Knight2006; Kjær and others, Reference Kjær2006; Piotrowski, Reference Piotrowski and Knight2006, Weaver and others, Reference Weaver, Arnaud and Bajc2011). The high-stress glaciotectonic regions provide escape routes for overpressurized water-laden sediments through associated glaciotectonic faulting and thrusting (Rijsdijk and others, Reference Rijsdijk, Owen, Warren, McCarroll and van der Meer1999; Le Heron and Etienne, Reference Le Heron and Etienne2005; Piotrowski, Reference Piotrowski and Knight2006; Phillips and Merritt, Reference Phillips and Merritt2008; van der Meer and others, Reference van der Meer, Kjaer, Kruger, Rabassa and Kilfeather2009). The upward narrowing configuration of the southeast verging dykes at Khalsar suggests that they were expelled upwards into the strata from below with stress probably from the northwest direction (Rijsdijk and others, Reference Rijsdijk, Owen, Warren, McCarroll and van der Meer1999; Vaughan and others, Reference Vaughan, England and Evans2014).
5.2. Role of permafrost
Ice-bonded permafrost has been considered necessary to increase the sediment cohesion and shear strength for the preservation of primary bedding within unconsolidated sediments in such heavily glaciotectonically deformed landforms (Kupsch, Reference Kupsch1962; Waller, Reference Waller, Elias and Mock2013; Vaughan and others, Reference Vaughan, England and Evans2014). The preservation of the primary banding and stratification in sand-rich graded diamicton (Dmg) and the prevalence of ductile deformation within the unsorted fine clay-rich diamicton (Dmm) has been attributed to the deformation at temperatures slightly below the pressure-melting point (Waller and others, Reference Waller, Phillips, Murton, Lee and Whiteman2011). This supports the prevalence of permafrost conditions at the glacial toe and in the entrapment of large megablock. The well-defined brittle fault gouge with slickensides within the diamicton at the Khalsar landform represents the basal displacement plane (Figs 4b, 7a, 9). The brittle failure is reported to be the dominant mode of glaciotectonic deformation in frozen sediments during glaciotectonism under glacial stress (Evans, Reference Evans, Elias and Mock2013). The presence of deformed and undeformed strata above and below the fault gouge also supports the brittle fault zone as the main translational thrust plane. The depth of the deformation surface, above which strata are displaced and deformed, and below which no deformation took place represents a décollement surface and is generally marked by a weak lithology or the lower limit of permafrost (Aber and Ber, Reference Aber, Ber, Singh, Singh and Haritashya2011). The permafrost at the glacial toe or snout restricts the drainage from subglacial aquifers and creates very high pore-water pressures at the base necessary for an ideal décollement along which displacement occurs (Mooers, Reference Mooers1990; Boulton and Cuban, Reference Boulton and Cuban1995; Boulton and others, Reference Boulton, Cuban and van Gijssel1995; Waller, Reference Waller, Elias and Mock2013). Brittle failure in coarser sediments cemented by frozen pore-water (pore ice) is reported at temperatures of <−0.5°C in sand (Waller and others, Reference Waller, Murton and Kristensen2012). Therefore, the presence of a well-developed fault gouge at the Khalsar site possibly indicates temperatures below −0.5°C, thereby promoting brittle failure. However, the diamicton dykes, with large boulder-sized clasts, testify to the presence of subglacial water with very high pore-water pressure due to high stress or possibly the patchy nature of subglacial permafrost at some stage of movement (Rijsdijk and others, Reference Rijsdijk, Owen, Warren, McCarroll and van der Meer1999). Further, the presence of clastic dykes within the decomposed granite indicates sufficiently high-glacial stress which might have exceeded the frozen rock's high shear strength, resulting in frictional heat release which inhibits the existence of continuous thick subglacial permafrost (van der Wateren, Reference van der Wateren1985; Hart and others, Reference Hart, Hindmarsh and Boulton1990).
The diamicton of the Khalsar landform site is characterized by prominent ductile deformation and thrusting. The ductile shear and thrust zones exhibit fault imbrication and tapering of gravel-rich diamicton (Dmm) within decomposed granite (Figs 9d, e, 10, 11). Under frozen conditions, the imbrication by thrusting is more reported in coarse diamicton clasts than in finer material (Kundic and Hanson, Reference Kundic and Hanson2006). The thin contact zone of overlying granite and underlying Quaternary diamicton at places shows high-strain cataclastic deformation and mixing between the two units which has resulted in the formation of the ductile mylonitic shear fabric of sigma-type and delta-type feldspar porphyroclasts with occasional stretching tails (Figs 11a–c). The porphyroclast in the mixed zone is surrounded and swerved by clay and silty clay groundmass. The presence of such high-strain ductile shear fabric within the surficial Quaternary unconsolidated sediments is unreasonable under low temperature normal orogenic conditions. However, such structures are possible and have been reported in fine clay-sized material under high-pressure frozen and/or fluidized thin skin glaciotectonic conditions (Burke and others, Reference Burke, Phillips, Lee and Wilkinson2009). Under permafrost conditions, pore ice cements the sand bodies as rigid bodies and concentrates the deformation within finer-grained diamicton because of its significant liquid water content and ductile rheology (Waller and others, Reference Waller, Phillips, Murton, Lee and Whiteman2011). Therefore, the rheological contrast between the frozen rigid sand-rich sediments, smaller granite rafts and the surrounding ductile fine clay-rich frozen diamicton with significant liquid water resulted in the ductile mylonitic deformation in finer diamicton. The ductile deformation zone within glacially deformed terrain constitutes the frozen glaciotectonite (Waller and others, Reference Waller, Murton and Whiteman2009). The thrusting and ductile mylonite fabric in the mixed mylonitic contact zone of diamicton and granite indicates very high-stress conditions. The ductile deformation in coarse-grained sediment has been related to the advancing glacial-induced pervasive deformation of the sediments and the resultant glaciotectonite melange (Vaughan and others, Reference Vaughan, England and Evans2014). However, the presence of upward narrowing diamicton dykes and change in deformation from brittle to ductile upwards indicates the incorporation of pore-water and may be related to the increase in subglacial stress, friction or geothermal heat and the corresponding increase in temperature up to pressure-melting point (Mooers, Reference Mooers1990; Dyke, Reference Dyke1993; Waller and others, Reference Waller, Murton and Kristensen2012; Vaughan and others, Reference Vaughan, England and Evans2014). The increase in pressurized meltwater beneath glaciers reduces the sediment shear strength (greater dilation) by lubricating the weak sedimentary beds and instigating ductile deformation in sediment (Twiss and Moores, Reference Twiss and Moores1992; Waller and others, Reference Waller, Murton and Kristensen2012). The diamicton and granite at the mixed mylonitic contact zone show a higher concentration of finer material (clay, silty clay) relative to the bigger clasts (Figs 11a–c). The partially frozen sediment slurry of ice, water and sediments under sub-zero temperatures must have undergone ductile deformation under higher glacial stress, with a much pronounced effect on fine-grained sediments at the mixed contact zone. The frictional heat release along the décollement surface during the transportation of mega-raft may change the subglacial thermal regime above the pressure-melting point to a warm-based regime. The direct juxtaposition of ductile and brittle deformation in diamicton, having coarser gravel size to fine clay sediments, points to the existence of warm permafrost (Waller and others, Reference Waller, Murton and Whiteman2009). Many researchers suggest that the warm permafrost is characterized by temperatures below, but close to, the melting point that permits relatively high amounts of water to exist within fine-grained sediment, possibly down to −7°C in very fine-grained clays (Tsytovich, Reference Tsytovich1975; Waller and others, Reference Waller, Murton and Kristensen2012; Vaughan and others, Reference Vaughan, England and Evans2014). The existence of very large raft, mylonitic glaciotectonite within fine diamicton and clastic dykes indicate the existence of both permafrost and meltwater conditions. Therefore, the polythermal regime is suggested, where permafrost may have existed in snout and proglacial portions which assisted in the propagation of décollement within the frozen unconsolidated diamicton and also in the entrapment of very large granite megablock to the base of the glacier. However, the increase in subglacial pore-water pressure behind and under the entrapped rock mass may have assisted in the extensive transportation of the glaciotectonic megablock. Further, the change and spatial variation of the glacial thermal regime during the entrapment, transport and emplacement of mega-raft cannot be ruled out, thereby, supporting the polythermal regime.
5.3. Origin and significance of rafts and megablocks
The entrainment of the large granite megablock can be attributed to both the high subglacial pore-water pressures by permafrost conditions at the glacier snout and also by a topographic obstruction within the pre-existing terrain (Schott, Reference Schott1933; Moran and others, Reference Moran, Clayton, Hooke, Fenton and Andriashek1980). The frozen conditions for sediments and/or bedrock up to the base of the glacier have been suggested for the transportation of detached intact glaciotectonic thrust blocks/megablocks or rafts (Clayton and Moran, Reference Clayton, Moran and Coates1974; Banham, Reference Banham, Wright and Moseley1975; Bluemle and Clayton, Reference Bluemle and Clayton1984; Ruszczynska-Szenajch, Reference Ruszczynska-Szenajch1987; Aber, Reference Aber and Croot1988). However, the transportation of unfrozen unconsolidated thrust-blocks by the fluid flow along the décollement surfaces has also been suggested (Moran and others, Reference Moran, Clayton, Hooke, Fenton and Andriashek1980; van der Wateren, Reference van der Wateren1985; Broster and Seaman, Reference Broster and Seaman1991; Aber and Ber, Reference Aber and Ber2007; Benediktsson and others, Reference Benediktsson2008; Phillips and Merritt, Reference Phillips and Merritt2008; Benn and Evans, Reference Benn and Evans2010; Phillips and others, Reference Phillips2017; Vaughan-Hirsch and Phillips, Reference Vaughan-Hirsch and Phillips2017). The topographic hindrance and the permafrost subglacial and proglacial setup may have caused the freezing of granite to the overriding glacier (Burke and others, Reference Burke, Phillips, Lee and Wilkinson2009). However, the geothermal gradient in very thick and large glaciers under frozen conditions increases the pore-water pressure, which may trigger the glacier movement (Boulton and others, Reference Boulton, Dobbie and Zatsepin2001). However, the coupling between large glacier and underlying frozen rigid rock under overpressurized hydrodynamic conditions may result in the plucking or beheading of the latter uneven topographic obstruction (Stalker, Reference Stalker1976; Aber, Reference Aber1985; Aber and Ber, Reference Aber and Ber2007). Thus the entrainment and transportation of a large granitic mega-raft requires its attachment to the base of a glacier under frozen conditions, supporting a prolonged cold thermal regime. The blocking of subglacial meltwater by mega-raft and frozen snout conditions may cause the movement of the glacier and subsequently the development of décollement at the base of mega-raft, snout and proglacial diamicton under permafrost conditions. The increase in pressurized meltwater enhances the ability of glaciers to detach and transport glaciotectonic thrust blocks and rafts along structural surfaces (e.g. Boulton and others, Reference Boulton, Dent and Morris1974, Reference Boulton, Dobbie and Zatsepin2001; Boulton and Cuban, Reference Boulton and Cuban1995; Hiemstra and van der Meer, Reference Hiemstra and van der Meer1997; Rijsdijk and others, Reference Rijsdijk, Owen, Warren, McCarroll and van der Meer1999; Phillips and Auton, Reference Phillips and Auton2000; Khatwa and Tulaczyk, Reference Khatwa and Tulaczyk2001; Baroni and Fasano, Reference Baroni and Fasano2006; Phillips and others, Reference Phillips, Merritt, Auton and Golledge2007, Phillips and others, Reference Phillips2018; van der Meer and others, Reference van der Meer, Kjaer, Kruger, Rabassa and Kilfeather2009; Sole and others, Reference Sole2011; Evans, Reference Evans2018). Therefore, based on well-preserved features as discussed, a subglacial transportation of the Khalsar landform as glaciotectonic nappe, under frozen to high-pressurized meltwater conditions is suggested. The stress propagation to the foreland seems very limited as indicated by the lack of significant folds, and the absence of stacking of multiple thrust sheets, slices and duplexes. The absence of such features does not support the exclusive proglacial environment. Also, the hill–hole pair feature has not been found in the area.
5.4. Origin of the Khalsar landform
The Khalsar landform has been considered lacustrine, a moraine deposit and rock avalanches, by different researchers (Phartiyal and Sharma, Reference Phartiyal and Sharma2009; Dortch and others, Reference Dortch, Owen and Caffee2010; Scherler and others, Reference Scherler2014; Ganju and others, Reference Ganju, Nagar, Sharma, Sharma and Juyal2018). However, the present study reveals that the unconsolidated clastic sedimentary strata overlain by decomposed granite cannot be related to the lacustrine or the rock avalanche. Further, the superimposition of a very large granite body over the diamicton sediments along with the presence of structural deformation features supports its glaciotectonic origin. Dortch and others (Reference Dortch, Owen and Caffee2010) considered the Khalsar landform as lateral moraine deposits of northwest flowing Shyok valley glacier. However, the deformational fabrics indicate top to the south/southeastward shear sense for the landform. The top to south shear sense is consistently observed in the Khalsar landform reflected by the tectonic alignment of diamicton pebbles, south verging convolutions or noses of diamicton (thrust propagation folds), convex top shear zones with flat base, south verging and tapering diamicton filled hydrofracture and slickensides (Figs 9b, d, e, 10). The uniform southeastward shear sense is attributed to the subglacial deformation by an advancing glacier from the northwest. Few upward narrowing clastic dykes verging towards the southeast support the southeastward subglacial stress which resulted in the hydrofracturing of overlying granite (Fig. 10b). The south to southeastward shear sense indicates that the compression or stress direction was coming from northwest, opposite to the flow direction of Shyok Valley Glacier. The extension of the Siachen Glacier along the Nubra valley has been reported beyond its confluence within the Shyok valley (Dortch and others, Reference Dortch, Owen and Caffee2010). We believe that the main and only possible glacial source for south to southeastward compression in the Khalsar glaciotectonic landform may be linked to the movement of the northwest to southeast flowing Siachen Glacier. The Siachen Glacier is interpreted to have formerly advanced along the entire Nubra valley in the northwest to southeast trend up to its confluence and at least further southeastwards up to the present reported landform per its northwest to southeast trajectory. However, earlier studies have reported the further extension of the Siachen Glacier beyond the Nubra and Shyok confluence taking a west-northwest downstream trend of the main trunk valley (Shyok valley) up to Hundar (Dortch and others, Reference Dortch, Owen and Caffee2010). However, the Khalsar glaciotectonic nappe is further southeastwards from the confluence ~5 km upstream in the Shyok valley. Therefore, it is presumed that the advancing Siachen Glacier must have pushed the Khalsar glaciotectonic nappe southeastwards in the upstream direction of the Shyok valley. The Khalsar glaciotectonic landform, being very large and rigid, could not have been diverted towards the west-northwest downstream direction and therefore, adopted the straight southeastward trajectory of the Siachen Glacier. Further, the role of fast advancing glaciers (surging glaciers) in extensive glaciotectonism, soft bed deformation, and glacial thrusting and overriding of marginal thick sediment sequences (till) under high-pressure subglacial water is well reported (Croot, Reference Croot and Croot1988; Sharp, Reference Sharp1988; Boulton and others, Reference Boulton1996; Truffer and others, Reference Truffer, Harrison and Echelmeyer2000; Fowler and others, Reference Fowler, Murray and Ng2001; Aber and Ber, Reference Aber, Ber, Singh, Singh and Haritashya2011). Such glacial surging results in a combination of brittle (faulting) and ductile (folding) deformation styles (Benediktsson and others, Reference Benediktsson2008). Therefore, it may also be possible that the advancement of the Siachen Glacier was very fast, thereby, adopting the easier southeastward linear trajectory rather than taking the west-northwest sharp bend.
6. Proposed glaciotectonic model for Khalsar landform
A glaciotectonic model has been developed for the origin of the Khalsar glaciotectonic landform (Fig. 12). The model discusses four main stages (a, b) preglacial fluvial environment in the Khalsar area and the cold glacial and frozen proglacial environment in the upstream of the Nubra valley. (c, d) The frozen to subglacial pore-water conditions are responsible for the brittle and ductile deformation in the subglacial and proglacial environments. The progress of brittle décollement surface with fault gouge over fluvial sediments, presence of undeformed sediments underlying the fault gouge and preservation of the primary fabric of sediments under the décollement surface indicate the permafrost proglacial environmental conditions were prevailing during the advancement of the glacier. (e) The emplacement and halt of glaciotectonic granite nappe over fluvial sediments under permafrost conditions and subsequent freeze–thaw cycles by increased water from the blocked Shyok River may have caused the extensive shattering and decomposition of granite block. (f) The glacier recession uncovers the undulatory granite block of glaciotectonic nappe and exposes it for aeolian erosional activity.

Figure 12. Glaciotectonic model proposed for the origin of the Khalsar landform. (a) An advancing Siachen Glacier flows over the uneven granite topography from the northwest to southeast direction, (b) the advancing glacier under high-stress plucks the large rock from the base, and stiffness at the base results in the faulting, (c) the advancing glacier along with plucked granite overrides the successive till/diamicton sediments and deforms them, with deformation signatures pointing towards southeast, (d) the glacier, allochthonous granite and caught up rafts and diamicton are thrusted over the fluvial sedimentary units of Shyok River, possibly under frozen conditions, (e) the glacier and allochthonous body is emplaced over the fluvial sedimentary units of Shyok River and Khardung basalt along a basal fault zone, representing thin skin glaciotectonic décollement surface, below which there is no deformation, (f) the retreat or diversion of the Siachen Glacier and termination of permafrost conditions expose this transported glaciotectonic landform in its present form. Under changing climatic conditions, the landform experiences new geomorphological changes and presently undergoes aeolian activity and grussification, (g–k) field photographs supporting the proposed glaciotectonic model for the origin of Khalsar landform, (g) field photograph of decomposed granite with clastic diamicton dyke on the left side, (h) thin glaciotectonite (GT) zone of mixed decomposed granite (DGR) and diamicton (Dmm(s)) units showing ductile deformation, (i) fault gouge (FG) at the contact between undisturbed underlying sand bed (Sh) and deformed overlying diamicton (Dmm(s)), (j) fused contact of overlying decomposed granite (DGR) and underlying diamicton (Dmm(s)) units, (k) graded and stratified diamicton (Dmg/Dms) and unsorted brecciated diamicton (Dmm(s)) underlying the decomposed granite as detailed in lithological log-2.
The glaciotectonic model proposed for the Khalsar glaciotectonic landform is elaborated in six main steps as depicted in Figure 12.
(a) The Siachen Glacier formerly flowing along the entire Nubra valley in the northwest to southeast direction is assumed to have advanced over an undulating or uneven rocky topography. The ice and glacial meltwater deposited the diamicton sediments at the snout. The diamicton sediments became younger towards the southeast direction. The topographic hindrance in the Nubra valley slowed the glacier movement and caused the freezing of marginal subglacial and proglacial environments with underlying granite exposure. The cold thermal regime at the marginal subglacial environment caused the freezing of granite under the overriding glacier. At the same time, a fluvial environment existed in the Khalsar area, where the deposition of the boulder (Gm) and Sand (Sh) occurred.
(b) The stress due to the weight and very extensive nature of the Siachen Glacier resulted in the plucking or beheading of a large granite megablock from the basal uneven frozen topographic surface and was transported en-mass as mega-raft. The transport of highly competent granite megablock under subglacial frozen conditions formed a décollement surface thrusting over the diamicton. The rock being granitic and frozen in nature had enough competency to override the diamicton sediments at successive snout locations. Further, the increase of glacial mass and frictional heat release by faulting also caused subglacial melting in some areas which increased the subglacial pore-water pressure and may have reduced the subglacial permafrost in some portions. The frictional heat release continuously increased the pore-water pressure and meltwater along the décollement plane, which increased the glacier movement including the entrapped megablock. This caused the brittle décollement surface to proceed further southeastwards under permafrost proglacial conditions.
(c) The transport of megablock under frozen subglacial conditions caused crushing and pulverization of diamicton in the form of fault gouge. The southeastward movement of the Siachen Glacier and transport of entrapped granite megablock caused the ductile deformation in the clay matrix-dominated diamicton under permafrost conditions. The coupling between the rigid granite and rheologically ductile diamicton under frozen to sub-frozen marginal glacier setting and under sufficient subglacial hydrodynamic conditions near the pressure melting point caused the ductile deformation just above the décollement surface at the interface of granite and diamicton. The movement of very large glacier under frozen to high pore-water subglacial stress conditions, therefore, indicates a change in thermal regime from cold-based to polythermal which caused fracturing and decomposition of granite megablock.
(d) The advancement of the Siachen Glacier in southeast trajectory resulted in the thrusting of granite allochthonous body over the successive younger diamicton and fluvial sediments of Shyok River at the Shyok–Nubra confluence near the Khalsar village under permafrost conditions, as indicated by the preservation of undeformed primary fabric within fluvial sediments. The underlying boulder (Gm) and sand (Sh) beds indicate that the area around Khalsar was under the fluvial environment before the glaciotectonic thrusting of the granite megablock. The transportation and en-route deformation resulted in the development of southeasterly oriented deformation fabric and glaciotectonic rafts.
(e) Its further advancement in the southeast direction stopped once the glaciotectonic body abutted against the Khardung basalt hills, thereby emplacing the glaciotectonic landform at its present location near Khalsar. This could also have dammed the Shyok River and increased the subglacial water, resulting in repeated freeze and thaw cycles in the highly jointed and fractured granite megablock.
(f) The retreat of the Siachen Glacier from the area uncovered the glaciotectonic landform as an allochthonous nappe over fluvial sediments of the Shyok River. The resultant glaciated landform revealed a continuous wavy surface with features of roche moutonnée and drumlins. The area falling in one of the highest mountain belts of the world has a highly arid climate, which allows cold aeolian conditions to modify the surface of glaciotectonic nappe, leaving behind the modified mounds of highly jointed to disintegrated granite exposures, aeolian granitic deflation rocks and desert pavement surface.
Multiple glaciotectonism episodes may have taken place in the formation and modification of the Khalsar landform, which is beyond the scope of the present study. Further, the deposition of a large glaciotectonic megablock might have resulted in the blocking of the Shyok River and contributed to the formation of a lake upstream, as indicated by the lacustrine sediments further upstream of the Shyok River.
7. Conclusion
The present study is the first report on glaciotectonism in Extra Peninsular India and reveals the thin skin glaciotectonic nappe origin for the Khalsar landform based on: (1) the presence of diamicton over fluvial sediments and under decomposed granite, (2) superimposition of older decomposed granite over the Quaternary unconsolidated diamicton and fluvial sediments along a brittle fault gouge zone, (3) ductile shear fabric at the contact zone of diamicton and overlying decomposed granite, (4) the presence of diamicton-filled SSD structures and hydrofractures and (5) juxtaposition of underlying undisturbed unconsolidated fluvial and diamicton sediments with overlying deformed diamicton and granite strata along a brittle fault gouge-breccia zone.
The presence of clastic dykes and glaciotectonite ductile shear fabric in diamicton and decomposed granite indicates the subglacial deformation under frozen to sub-frozen and highly pressurized water conditions. The glaciotectonic deformational fabric of slickensides, dragged ductile fold noses, shear planes and ductile shear fabric with crude foliation in diamicton and granite consistently show southeastward orientation. The Siachen Glacier advancing from northwest to southeast is presumed to be the only glacial source for such southeastward orientation.
Data
The data that support the findings of this study are available within the article and can be available from the corresponding author upon reasonable request.
Acknowledgements
The authors acknowledge the Geological Survey of India for extending all the support for the fieldwork. The first author is grateful to Dr Riyaz Ah. Dar, University of Kashmir, Shri. Muzaffar Ah. Najar and Zahid Majeed, GSI, Srinagar, J&K, and Dr Shamim Ah. Dar, Banaras Hindu University for extending the support and technical suggestions. Last but not least, the authors highly appreciate the critical review and helpful comments of the Dr Richard Waller and another anonymous reviewer and scientific editor for improving the present paper. The findings in this paper are part of the project no. M4LSSI/NC/NR/SU-JK/2022/41454.
Author contributions
Abdul Qayoom Paul: investigation, data curation, methodology, conceptualization and writing – original draft. Harish Bahuguna: data curation, methodology, conceptualization and writing – original draft. Parveen Kumar: investigation, data curation and methodology.