Atherosclerosis is a progressive pathological disorder that often leads to CVD and cerebrovascular diseases. Despite improved pharmacological agents and changes in lifestyle, atherosclerosis is still a leading cause of mortality and morbidity in industrialized countries(Reference Braunwald1). This chronic inflammatory disease is driven by risk factors that cause oxidative and inflammatory mechanisms(Reference Kaperonis, Liapis, Kakisis, Dimitroulis and Papavassiliou2). Oxidative stress may trigger many cellular events, such as the inactivation of NO, oxidative modifications of DNA, proteins and lipids, and enhanced mitogenicity and apoptosis of vascular cells(Reference Muller, Rupec and Baeuerle3). Furthermore, oxidative stress can also activate or increase the expression of redox-sensitive genes, such as those coding for oxidized LDL receptors, adhesion molecules, chemotaxis factors, proinflammatory cytokines, regulators of cell cycle progression and matrix metalloproteinases (MMP)(Reference Wassmann, Wassmann and Nickenig4). Previous studies have also indicated that proinflammatory cytokines, such as TNF and IL-1, play an important role in the pathogenesis of atherosclerosis(Reference Zhu, Liao, Lin, Verna and Stemerman5, Reference Rahman, Kefer, Bando, Niles and Malik6).
The migration of smooth muscle cells (SMC) from the tunica media to the subendothelial region is a key event in the development and progression of many vascular diseases, including atherosclerosis and post-angioplasty restenosis(Reference Maeda, Kuzuya, Cheng, Asai, Kanda, Tamaya-mori, Sasaki, Shibata and Iguchi7). Activation of MMP may contribute to the pathogenesis of atherosclerosis by facilitating migration of SMC(Reference Jones, Sane and Herrington8). Analysis of human atherosclerotic lesions and advanced plaques has indicated an increased expression of MMP, especially the gelatinases MMP-2 and MMP-9, predominantly in vascular SMC(Reference Galis, Johnson, Godin, Magid, Shipley, Senior and Ivan9).
The pathogenesis of atherosclerosis also involves the transcription factor NF-κB, and its target genes(Reference Kutuk and Basaga10). NF-κB subunits form homo- and heterodimers, with the most prominent being the p50/p65 heterodimer. The dimer is retained in the cytoplasm in an inactive state through interaction with inhibitory protein κB(Reference Barnes and Adcock11). NF-κB is then rapidly activated in response to a variety of inflammatory and other stimuli that lead to the degradation of inhibitory protein κB(Reference De Martin, Hoeth, Hofer-Warbinek and Schmid12). Upon activation of NF-κB, a large number of genes are induced, including various inflammatory cytokines, adhesion molecules and MMP(Reference De Martin, Hoeth, Hofer-Warbinek and Schmid12–Reference Neish, Williams, Palmer, Whitley and Clollins14).
NF-κB activation, which is followed in vitro by elevation in free radical levels, was demonstrated to be inhibited by antioxidants such as gallates, cafeic acid, curcumin and others(Reference Murase, Kume, Hase, Shibuya, Nishizawa, Tokimitsu and Kita15–Reference Chan17). Carnosic acid (CA), a major phenolic constituent in rosemary (Rosmarinus officinalis) and sage (Salvia officinalis)(Reference Luis, Martin Perez and Valdes Gonzalez18, Reference Baskan, Oztekin and Erim19), has a typical O-diphenol structure (Fig. 1). Most diphenol compounds have been shown to have potent chain-breaking antioxidant activity(Reference Shahidi, Janitha and Wanasundara20–Reference del Bano, Lorente, Castillo, Benavente-Garcia, del Rio, Ortunto, Quirin and Gerard22). Previous studies have indicated that the extracts of rosemary and sage possess antioxidant, antimicrobial and anti-inflammatory effects(Reference Oluwatuyi, Kaatz and Gibbson23, Reference Moreno, Scheyer, Romano and Vpjnov24), and inhibit lipid absorption in man(Reference Ninomiya, Matsuda, Shimoda, Nishida, Kasajima, Yoshino, Morikawa and Yoshikawa25). However, the effect of CA on the molecular mechanism of MMP-9 expression in cultured SMC has not yet been elucidated. We hypothesized that TNF-α-induced MMP-9 expression was abolished by CA in human aortic smooth muscle cells (HASMC) via inhibition of transcription factor NF-κB binding activities. Therefore, we investigated the effects of CA on TNF-α-induced cell migration, the formation of intracellular reactive oxygen species (ROS), the translocation of NF-κB, and the activation and expression of MMP-9 in HASMC.

Fig. 1 The structure of carnosic acid.
Materials and methods
2,2-Diphenyl-1-picrylhydrazyl scavenging assay
The free radical scavenging effect was determined using the free radical generator 2,2-diphenyl-1-picrylhydrazyl (DPPH) proposed by Yamaguchi et al. (Reference Yamaguchi, Takamura, Matoba and Terao26). Briefly, the reaction mixture contained 500 μl CA (0–200 μmol/l) and 500 μl DPPH (0·5 mmol/l in methanolic solution). The radical scavenging activity of DPPH was evaluated by measuring the change in absorbance at 517 nm. Decoloration was plotted against the sample extract concentration in order to calculate the ic50 values (the amount of sample necessary to decrease the absorbance of DPPH by 50 %).
Trolox equivalent antioxidant capacity assay
The Trolox equivalent antioxidant capacity (TEAC) was determined according to the method of Miller et al. (Reference Miller, Rice-Evans, Davies, Gopinathan and Miller27). A value of 1 TEAC in a sample is defined as a concentration equivalent to 1 (mol/l Trolox, a water-soluble analogue of α-tocopherol.
Inhibition of LDL oxidation
LDL was isolated after ultracentrifugation as described previously(Reference Mackness, Durringtom, Converse and Skinner28). The LDL fraction was dialysed against a PBS buffer of pH 7·4 without EDTA in the dark, filtered through a 0·45 μm filter, stored at 4°C under nitrogen, and used within 24–72 h. The LDL was oxidized using classical copper-induced LDL auto-oxidation. Increasing concentrations of CA (approximately 0–10 μmol/l) dissolved in dimethyl sulphoxide were added to the incubation media. Incubations were carried out at 37°C to measure the formation of conjugated dienes. Briefly, LDL total cholesterol (0·9 mg/ml) was incubated in PBS in the presence of CuSO4 (50 μmol/l). After incubation, 150 μl EDTA (2 mmol/l) was added. A 100 μl portion of the mixture was then transferred to vials containing 0·9 ml 2-propanol. The precipitates were removed via centrifugation. The concentration of conjugated diene in the supernatant was determined by measuring the absorption at 234 nm.
Cell culture
HASMC were purchased from the Food Industry Research and Development Institute, Chin-Tsu, Taiwan (CCRC 60 293). They were maintained in Ham's F12K medium containing 10 % fetal bovine serum, 2 mmol/l l-glutamine, 1·5 g/l sodium bicarbonate, 10 mmol/l HEPES, 10 mmol/l (N-tris) hydroxymethy-2-aminoethanesulfonic acid, 0·05 mg/ml ascorbic acid, 0·01 mg/ml transferrin, 0·01 mg/ml insulin, 10 ng/ml sodium selenite and 0·03 mg/ml epidermal growth factor. All experiments were performed with HASMC in passages 21–31, which had been grown to 80–90 % confluence and made quiescent by serum starvation (0·1 % fetal bovine serum) for at least 24 h.
Cell viability assay using 3-(4,5-dimethylthiazol-2-yl)-2,5-diphenyl tetrazolium bromide
The cytotoxic effect of CA on HASMC was investigated using 3-(4,5-dimethylthiazol-2-yl)-2,5-diphenyl tetrazolium bromide (MTT) assay(Reference Chen, Lin, Chen, Ku and Chen29). The principle of this assay is that mitochondria dehydrogenase in viable cells reduces MTT to a blue formazan product. Briefly, the cells were grown in ninety-six-well culture plates at a density of 1 × 104 cells per well in F-12K culture medium and incubated with various concentrations of CA for 24 h. Then, 10 μl MTT (5 mg/ml) was added to each well and incubation was allowed to continue at 37°C for an additional 4 h. The medium was then carefully removed, so that the formazan crystals that had formed were not disturbed. Dimethyl sulphoxide (100 μl), which solubilizes formazan crystals, was added to each well, and absorbance of the solubilized blue formazan was measured at an optical density of 590 nm using the μQuant Microplate Spectrophotometer (Bio-Tek, VT, USA). All determinations were performed according to three individual experiments. Each individual experiment included two duplicated experiments. The data are shown as means and standard deviations, as a percentage of the control.
Examination of matrix metalloproteinase-9 by gelatin zymography
MMP-9 activity in conditioned medium of cultured HASMC was analysed by substrate-gel electrophoresis (zymography) using SDS–PAGE (10 %) containing 0·1 % gelatin. Substrate gel zymographic quantification of the activity of MMP-9 was performed with a Mini-Protein II apparatus from Bio-Rad, according to a method described previously(Reference Demeule, Brossard, Page, Gingras and Beliveau30). Cells were grown to sub-confluence, rinsed with PBS, and then incubated in serum-free medium for 24 h. Equal volumes of samples of conditioned cell culture medium were mixed with sample buffer containing 62·5 mmol/l 2-amino-2-hydroxymethyl-1,3-propanediol hydrochloride (Tris–HCl) (pH 6·8), 10 % glycerol, 2 % SDS and 0·00 625 % (w/v) bromophenol blue, loaded on to the gel and separated by electrophoresis. Thereafter, gels were washed three times for 30 min at room temperature in buffer (50 mmol/l Tris–HCl, pH 8·0, 5 mmol/l CaCl2, 0·02 % NaN3 and 2·5 % Triton X-100) and incubated for 18 h at 37°C with the same buffer, minus Triton X-100. Gels were stained with Coomasssie Brilliant Blue R-2500 (0·1 %) and destained in 5 % methanol and 7 % acetic acid. Gelatinolytic activity was represented as a clear band on a blue background.
Western blot analysis for the expression of matrix metalloproteinase-9
HASMC were treated with various concentrations of CA in the presence of 100 ng/ml TNF-α. Cellular lysates were prepared in a lysis buffer containing 10 mmol/l Tris–HCl (pH 8), 0·32 mol/l sucrose, 5 mmol/l EDTA, 1 % Triton X-100, 2 mmol/l 1,4-dithio-d, l-threitol and 1 mmol/l phenylmethylsulphonyl fluoride. The cells were disrupted and extracted at 4°C for 30 min. After centrifugation at 13 000 rpm for 15 min, the cell lysate was obtained as the supernatant. Protein concentrations were measured using the Bradford assay. Total protein (20 μg) was subjected to SDS–PAGE (10 %) and blotted on polyvinylidene difluoride membranes. Soaking the membrane in PBS–Tween 20 buffer containing 50 g/l non-fat milk blocked non-specific binding. The membrane was incubated with monoclonal mouse anti-human β-actin (1:1000) and polyclonal rabbit anti-human MMP-9 (1:1000). Subsequently, the membrane was incubated with sheep anti-mouse IgG antibody (1:5000) and goat anti-rabbit IgG antibody (1:5000) (Abcam, Cambridge, UK). The protein levels were determined using enhanced chemiluminescence detection reagents (Upstate, CA, USA) and high-performance chemiluminescence film (Amersham, IL, USA). Incubation with mouse anti-human β-actin antibody was also performed as an internal control. Results were quantified with a scanning densitometer using an image analysis system with software.
ELISA-based NF-κB assay
In addition to gel-shift assays, an ELISA-based kit was used for quantitative detection of NF-κB activity. Nuclear protein extracts of HASMC were prepared using a Trans AM Nuclear Extract Kit (Trans AM, CA, USA). For each sample, 20 μl of nuclear extracts (5 μg protein) were used according to the manufacturer's instructions. Nuclear extracts were incubated in the oligonucleotide-coated wells for 60 min. Where indicated, a competitor for NF-κB binding (NF-κB wild-type consensus oligonucleotide) was added in molar excess prior to the probe. The wells were then washed and incubated with the primary antibodies for p50 and p65 for 60 min. After incubation with a horseradish peroxidase-conjugated secondary antibody, a substrate was added to produce a colour reaction; the reaction was quantitated by a μQuant Microplate Spectrophotometer (Bio-Tek). The absorbance was read at 590 nm and the blank was subtracted from all measurements.
Cell migration assay
The invasion of vascular SMC through the extracellular matrix was determined by a commercial cell invasion assay kit (Chemicon, CA, USA)(Reference Bedoui, Oak, Anglard and Schini-Kerth31). HASMC (1·5 × 105 cells/300 μl) were resuspended in conditioned medium collected after pretreatment with CA and TNF-α-treated cells for 23 h, and added to the upper components of the migration chamber. Then, 500 μl of the same conditioned medium was added to the lower compartment of the migration chamber. Cells without TNF-α-treated conditioned medium served as the control. The migration chambers were incubated at 37°C for 24 h in 5 % CO2. After incubation, the inserts were removed from the wells, and the cells on the upper side of the filter were removed using cotton swabs. The filters were fixed, and stained according to the manufacturer's instructions. Then, 100 μl of the dye mixture was transferred to a ninety-six-well plate, and the optical density was measured at 560 nm.
Measurement of intracellular reactive oxygen species
HASMC were pretreated with 10 and 20 μmol/l CA for 1 h and induced by TNF-α (100 ng/ml) for 23 h. The cells were then incubated for 30 min with 10 μmol/l 2,7-dichlorofluorescein diacetate, which is converted to dichlorofluorescein by intracellular esterase. The latter was then oxidized by ROS to the highly fluorescent dichlorofluorescein. The fluorescence of each dish was immediately analysed at an excitation wavelength of 485 nm and an emission wavelength of 528 nm by an FL × 800 microplate fluorescence reader (Bio-Tek)(Reference Kim, Kim, Koh, Ho and Lee32). All measurements were at least triplicated.
Statistical analyses
Results are shown as means and standard deviations. Statistical analyses of MTT were performed using one-way ANOVA followed by Dennett's test; the other analyses were performed using one-way ANOVA followed by Duncan's multiple range test. A value of P < 0·05 was considered statistically significant.
Results
The antioxidative capacity of carnosic acid in vitro
The free radical scavenging effect of CA was determined using the free radical generator DPPH; the ic50 of the DPPH assay was 35·9 (sd 1·7) μmol/l (Table 1). The LDL oxidation assay was inhibited by CA and the ic50 of the inhibited LDL oxidation was 5·63 (sd 0·19) μmol/l (Table 1). The TEAC assay is based on the reduction of the 2, 2′-azino-bis (3-ethylbenzthiazolien-6-sulfonic acid) radical cation by antioxidants; the TEAC value of CA was 5·75 (sd 0·49) (Table 1).
Table 1 Antioxidative capacity of carnosic acid in vitro *
(Mean values and standard deviations for three determinations)
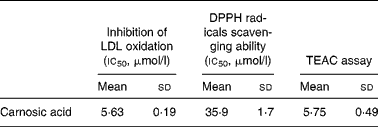
DPPH, 2,2-diphenyl-1-picrylhydrazyl; TEAC, Trolox equivalent antioxidant capacity.
* For details of procedures, see Materials and methods ic50 values were obtained from the concentration response curves.
Concentrations of carnosic acid for human aortic smooth muscle cells
The cytotoxicity of CA on HASMC was evaluated using the MTT assay. CA had a dose-dependent cytotoxic effect on HASMC. After 24 h incubation with 0, 10, 20, 30, 50 and 75 (mol/l CA, cell viability was 100, 94·9 (sd 1·2), 92 (sd 0·5), 84·9 (sd 0·6), 79·7 (sd 1·6) and 67·7 (sd 2·6) %, respectively, of the control level. Therefore, according to the MTT assay, we chose 10 and 20 μmol/l CA to perform all of the following experiments.
Carnosic acid prevents TNF-α-induced activation of matrix metalloproteinase-9 in human aortic smooth muscle cells
The effect of CA on TNF-α-induced MMP-9 activation was analysed by gelatin zymography. As shown in Fig. 2, MMP-9 secretion was markedly induced by TNF-α, and suppressed by CA pretreatment. The 20 μmol/l CA treatment was more effective at inhibiting the activation of MMP-9 than the 10 μmol/l CA treatment.

Fig. 2 Effect of carnosic acid (CA) on the matrix metalloproteinase-9 (MMP-9) activity of TNF-α-induced human aortic smooth muscle cells (HASMC). HASMC were pretreated with 10 and 20 μmol/l CA for 1 h, and induced by TNF-α (100 ng/ml) for an additional 23 h. (A), The activation of MMP-9 was assessed by gelatin zymography. (B), Densitometric analysis was conducted with image analysis system software to quantify gelatin zymography data. Values are means with their standard deviations depicted by vertical bars (n 3). Each individual experiment included two duplicated experiments. a–d Mean values with unlike letters were significantly different (P < 0·05). C, control without TNF-α or CA; CA10, 10 μmol/l CA; CA20, 20 μmol/l CA; TNF, TNF-α.
Carnosic acid suppresses TNF-α-induced matrix metalloproteinase-9 expression in human aortic smooth muscle cells
The effect of MMP-9 expression by CA in vascular SMC was assessed by Western blot. HASMC were pretreated with 10 and 20 μmol/l CA for 1 h, and induced by TNF-α (100 ng/ml) for 23 h. MMP-9 expression was markedly induced by TNF-α, and suppressed by CA pretreatment (Fig. 3).

Fig. 3 Carnosic acid (CA) inhibits the protein expression of matrix metalloproteinase-9 (MMP-9) in human aortic smooth muscle cells (HASMC). HASMC were pretreated with 10 and 20 μmol/l CA for 1 h, and induced by TNF-α (100 ng/ml) for an additional 23 h. The expression of MMP-9 was assessed by Western blot analysis. (A), Representative Western blot showing MMP-9 protein levels in cell lysates (top) and β-actin (bottom). (B), Densitometric analysis was conducted with image analysis system software to quantify Western blot data. Values are means with their standard deviations depicted by vertical bars (n 3). Each individual experiment included two duplicated experiments. a,b,c Mean values with unlike letters were significantly different (P < 0·05). C, control without TNF-α or CA; CA10, 10 μmol/l CA; CA20, 20 μmol/l CA; TNF, TNF-α.
Carnosic acid suppresses nuclear translocation of NF-κB p50 and p65 in TNF-α-induced human aortic smooth muscle cells
To determine whether the inhibitory effect of CA on the TNF-α-induced expression of MMP-9 is medicated via NF-κB, we measured the nuclear translocation of p50 and p65 of the NF-κB family. Treatment of TNF-α (100 ng/ml) for 23 h enhanced the nuclear translocation of p50 (Fig. 4) and p65 (Fig. 5). Pretreatment of HASMC with 10 and 20 μmol/l CA prior to TNF-α stimulation significantly prevented the nuclear translocation of p50 and p65. In Fig. 4, the 20 μmol/l CA treatment was more effective at decreasing nuclear translocation of NF-κB p65 than the 10 μmol/l CA treatment.

Fig. 4 Effect of carnosic acid (CA) on TNF-α-induced activation of NF-κB p50 in human aortic smooth muscle cells (HASMC). HASMC were pretreated with 10 and 20 μmol/l CA for 1 h and induced by TNF-α (100 ng/ml) for 23 h. Nuclear extracts were prepared and analysed for activation of the NF-κB family; 5 μg nuclear protein were used in each experiment. Values are means with their standard deviations depicted by vertical bars (n 3). Each individual experiment included two duplicated experiments. a,b,c Mean values with unlike letters were significantly different (P < 0·05). C, control without TNF-α or CA; CA10, 10 μmol/l CA; CA20, 20 μmol/l CA; TNF, TNF-α.

Fig. 5 Effect of carnosic acid (CA) on TNF-α-induced activation of NF-κB p65 in human aortic smooth muscle cells (HASMC). HASMC were pretreated with 10 and 20 μmol/l CA for 1 h and induced by TNF-α (100 ng/ml) for 23 h. Nuclear extracts were prepared and analysed for activation of the NF-κB family; 5 μg nuclear protein were used in each experiment. Values are means with their standard deviations depicted by vertical bars (n 3). Each individual experiment included two duplicated experiments. a–d Mean values with unlike letters were significantly different (P < 0·05). C, control without TNF-α or CA; CA10, 10 μmol/l CA; CA20, 20 μmol/l CA; TNF, TNF-α.
Carnosic acid suppresses TNF-α-induced human aortic smooth muscle cell migration
As shown in Fig. 6, the migration of HASMC increased after treatment with TNF-α relative to that of TNF-α-untreated control cells. The stimulatory effect of TNF-α significantly decreased after CA pretreatment. The 20 μmol/l CA treatment was more effective on decreasing the level of HASMC migration than the 10 μmol/l CA treatment.

Fig. 6 Effect of carnosic acid (CA) on migration of human aortic smooth muscle cells (HASMC) induced by TNF-α treatment. HASMC (1·5 × 105 cells/300 μl) were resuspended in a conditioned medium after treatment with TNF-α for 23 h, and added to the upper components of the migration chamber in the presence of 10 and 20 μmol/l CA. Values are means with their standard deviations depicted by vertical bars (n 3). Each individual experiment included two duplicated experiments. a,b,c Mean values with unlike letters were significantly different (P < 0·05). C, control without TNF-α or CA; CA10, 10 μmol/l CA; CA20, 20 μmol/l CA; TNF, TNF-α.
Carnosic acid suppresses TNF-α-induced reactive oxygen species generation
The production of ROS was induced by TNF-α and decreased by CA. The 20 μmol/l CA treatment was more effective at reducing ROS generation than the 10 μmol/l CA treatment (Fig. 7).

Fig. 7 Effect of carnosic acid (CA) on TNF-α-induced reactive oxygen species (ROS) production in human aortic smooth muscle cells (HASMC). (A), Microphotograph of ROS production in HASMC without TNF-α or CA (a), with TNF-α (100 ng/ml) (b), with TNF-α (100 ng/ml) and 10 μmol/l CA (c), with TNF-α (100 ng/ml) and 20 μmol/l CA (d). (B), HASMC were pretreated with 10 and 20 μmol/l CA for 1 h and induced by TNF-α (100 ng/ml) for 23 h. Values are means with their standard deviations depicted by vertical bars (n 3). Each individual experiment included two duplicated experiments. a,b,c Mean values with unlike letters were significantly different (P < 0·05). C, control without TNF-α or CA; CA10, 10 μmol/l CA; CA20, 20 μmol/l CA; TNF, TNF-α.
Discussion
In the present study, we investigated the effect of CA on HASMC migration and TNF-α-induced MMP-9 activation. Gelatin zymography and Western blot assays revealed that CA lowered the level of secretion and protein expression of MMP-9, as well as suppressed the nuclear translocation of the NF-κB p50 and p65. In addition, CA effectively inhibited the TNF-α-induced migration of HASMC.
Sage (S. officinalis) and rosemary (R. officinalis) are Labiatae herbs commonly used in cooking and folk medicines around the world(Reference Masuda, Inaba and Takeda21). CA is a phenolic diterpene compound found in sage (2–5 mg CA/g sage) and rosemary (12–15 mg CA/g rosemary)(Reference Luis, Martin Perez and Valdes Gonzalez18, Reference Baskan, Oztekin and Erim19). It is a lipophilic antioxidant that scavenges singlet oxygen, hydroxyl radicals and lipid peroxyl radicals, thus preventing lipid peroxidation(Reference Shahidi, Janitha and Wanasundara20–Reference del Bano, Lorente, Castillo, Benavente-Garcia, del Rio, Ortunto, Quirin and Gerard22). In the present in vitro study, we found that CA could scavenge DPPH radicals, alkoxyl radicals (RO) and lipid peroxyl radicals (ROO) (Table 1). Furthermore, we found that CA has the ability to suppress TNF-α-induced intracellular ROS production (Fig. 7), and that its antioxidative ability is approximately 5–6-fold more potent than Trolox (Table 1).
The migration of SMC from the tunica media to the subendothelial region is a key event in the development and progression of atherosclerosis(Reference Maeda, Kuzuya, Cheng, Asai, Kanda, Tamaya-mori, Sasaki, Shibata and Iguchi7). MMP (MMP-2 and MMP-9) activity may contribute to the pathogenesis of atherosclerosis by facilitating the migration of SMC(Reference Jones, Sane and Herrington8). Although MMP-2 and MMP-9 have similar substrate specificities, the regulation of their expression is different. MMP-2 is constitutively expressed in several cell types, including SMC, and its expression is not induced by cytokines or growth factors. In contrast, MMP-9 can be induced by TNF-α in SMC(Reference Cho, Graves and Reidy33, Reference Galis, Muszynski, Sukhova, Simon-Morrissey, Unemori, Lark, Amento and Libby34). Therefore, we have investigated the effect of CA on TNF-α-induced SMC migration and activation of MMP-9. The present results indicate that the migration of HASMC is significantly induced by TNF-α and suppressed by 10 and 20 μmol/l CA pretreatment (Fig. 6). This inhibition of TNF-α-induced migration of HASMC is consistent with the inhibition of activation and expression of MMP-9 (Figs. 2 and 3). Similar results were seen when SMC were pretreated with other polyphenolic compounds, such as tea flavonoid epigallocatechin-3-gallate (20 μmol/l), quercetin (40 μmol/l) and other flavonoids(Reference Kim and Moon35–Reference Kris-Etherton and Keen37).
Further research beyond the scope of the present study is necessary to elucidate the mechanisms underlying the synergistic regulation of MMP secretion by cytokines. In the present study, we focused instead on defining the role played by the NF-κB transcription factor in the regulation of MMP in HASMC. A functional NF-κB site occurs in the proximal stimulatory region of the MMP-9 promoter(Reference Sato and Seiki38, Reference Fini, Bartlett and Matsubara39), and deletion of this site reduces up-regulation of reporter gene constructs in response to cytokines. Until now, however, it has been unclear as to the role NF-κB plays in the up-regulation of the endogenous MMP-9 gene. Bond et al. (Reference Bond, Chase, Baker and Newby40) have demonstrated that transient overexpression of inhibitory protien κBα in vascular SMC only partially impaired up-regulation of MMP-9, suggesting that NF-κB might play a simple permissive role. In the present study, CA reduced cytokine-induced expression of MMP-9 and prevented the nuclear translocation of p50 (Fig. 4) and p65 (Fig. 5) in HASMC. We suggest that inhibitory mechanisms of CA might interrupt a signalling cascade involving MMP-9 transcription-mediated activation of NF-κB.
Several studies have indicated that ROS are implicated in the activation of NF-κB(Reference Muller, Rupec and Baeuerle3). The current study showed that CA pretreatment in HASMC decreased the TNF-α-stimulated production of ROS (Fig. 7). Based on this result, we propose that the inhibitory effect of CA on MMP-9 expression and NF-κB activation may be due to its antioxidant and anti-inflammatory properties. Since atherosclerosis is a chronic inflammatory disease associated with increased oxidative stress in HASMC, it would be conceivable that the anti-atherogenic effects of CA might be caused by its antioxidative and anti-inflammatory properties.
In conclusion, CA effectively inhibited the TNF-α-induced migration of HASMC. The levels of ROS production, MMP-9 activation and expression, and nuclear translocation of NF-κB p50 and p65 were also all reduced by CA pretreatment. The present results led us to conclude that CA inhibits TNF-α-induced nuclear translocation of p50 and p65, thereby suppressing the activation and protein expression of MMP-9, resulting in decreased HASMC migration. Thus, CA may play an important role in the prevention of atherosclerosis.
Acknowledgements
This work was supported by the National Science Council of Taiwan (NSC 96-2320-B-309-004-MY2). There is no conflict of interest. All of the authors have contributed substantially to the manuscript. The authors would like to thank Margaret Chang for preparation of this manuscript.