Whey proteins are a by-product of cheese manufacturing. In recent years, these dietary proteins have gained considerable interest in relation to their health benefits, including reduction in energy intake and body weight, and improvement in insulin sensitivity( Reference Krissansen 1 , Reference Luhovyy, Akhavan and Anderson 2 ). However, more research is required to assess whether the effects of whey protein are dependent on macronutrient composition in the diet, as demonstrated previously for diets enriched with whey protein( Reference Pichon, Potier and Tome 3 ), as this will allow the formulation of diets with the optimum macronutrient combination that confers specific beneficial effects on human health. Furthermore, such analysis may also reveal the mechanisms underpinning the effects of these proteins.
We have previously shown that intake of 20 % energy from whey protein isolate (WPI) reduces weight gain and fat mass, and increases lean mass in mice fed a high-fat (45 % energy) diet( Reference McAllan, Keane and Schellekens 4 , Reference McAllan, Skuse and Cotter 5 ). In contrast, intake of a high whey protein content (above the 14–25 % adequate energy range) has been shown to influence energy balance in mice fed a low-fat diet (10 % energy from fat). Moreover, where an effect is found, the underlying mechanisms have not been elucidated. For instance, mice fed a diet containing 30 % energy from whey protein and 10 % energy from fat exhibited reduced energy intake and weight gain by 1 week compared with mice fed a similar diet but with either soya or gluten as the protein source( Reference Yu, South and Huang 6 ). A reduction in cumulative energy intake was observed in rats fed a diet containing 42 % energy from protein (26 % whey and 16 % albumin) between 5 and 10 weeks compared with those fed a similar diet but whey replaced by either albumin or soya protein( Reference Zhou, Keenan and Losso 7 ). In contrast, when energy from dietary protein was reduced to 15 or 25 %, with energy from fat being maintained at about 9 and 15 %, respectively, whey protein did not affect energy intake and body-weight gain( Reference Morifuji, Sakai and Sanbongi 8 , Reference Teixeira, Silva and Neves 9 ). Interestingly, the latter studies assessed food intake only for a period of 2 or 7 weeks, in comparison to the study on high whey protein, in which the reduction in energy intake occurred by week 5 and continued up to 10 weeks( Reference Zhou, Keenan and Losso 7 ). Thus, this discrepancy in the data could be attributed to the test duration, where a longer duration is required when an adequate proportion of energy derived from protein is used in test diets. Thus, the purpose of the present study was to assess whether WPI at 20 % energy in a diet containing 10 % energy from fat alters energy balance and, where there is an effect, to explore the underlying mechanisms. The study lasted for 15 weeks in consideration of the previous published data showing that whey protein (at an adequate range) did not affect energy balance up to 7 weeks( Reference Morifuji, Sakai and Sanbongi 8 , Reference Teixeira, Silva and Neves 9 ).
Materials and methods
Animals and diets
All procedures involving animals were approved by the University College Cork Animal Experimentation Ethics Committee (2011/005) and were licensed under the Cruelty to Animals Act 1876. Male C57BL/6J mice (Harlan), aged 3 weeks old, were singly housed and had ad libitum access to food and water throughout the trial. Mice were initially fed a diet containing 10 % energy from fat and 35 % energy from sucrose with 20 % energy from casein (LF-CAS, #D12450; Research Diets) during the acclimatisation period lasting for 3 weeks.
Experimental protocol
Following acclimatisation, singly housed mice were separated into two weight-matched dietary groups (n 8 animals per group) and provided with either the LF-CAS diet or a similar diet containing 10 % energy from fat and 35 % energy from sucrose with 20 % energy from whey protein isolate (LF-WPI, AlacenTM 895; NZMP) for a total of 15 weeks. Further details of the diet composition can be found in online Supplementary Table S1. Body weight and energy intake were measured weekly. In addition, at week 14, feeding behaviour and metabolic activity were measured using the TSE PhenoMaster System (TSE Systems GmbH) comprising eight test cages and one reference cage. Mice were housed individually in the test cages. After a 2 d acclimatisation period, metabolic parameters were measured on the 3rd day. Sensors recorded metabolic parameters in the cages sequentially, with each cage being analysed for 1 min, such that data collected over a 24 h period corresponded to intervals of 9 min for the eight test cages and one reference cage. Body composition was determined by NMR using a Bruker Minispec LF50H (Bruker Optics) at the end of the study, following a 6–8 h fast. Subsequently, mice were anaesthetised and blood was collected. Mice were then killed by cervical dislocation. Tissues of interest were excised and weighed. Small-intestinal luminal contents were removed by mechanical expulsion and tissue length was determined. Tissue was weighed, and 2 cm sections from the proximal and distal parts of the intestine that were 2 cm away from the start and end of the intestine, respectively, were excised for the analysis of gene expression in the duodenum and ileum. All tissues were immediately snap-frozen in liquid N2. Plasma and tissue samples were stored at − 80°C until analysis.
Biochemical analysis
Blood collected into EDTA-containing vacutainer tubes (BD) from anaesthetised mice (ketamine, 65 mg/kg and xylazine, 13 mg/kg) was treated with diprotin A and aprotinin, as described previously( Reference McAllan, Keane and Schellekens 4 ), to protect plasma peptides against proteolytic degradation. Plasma was isolated by centrifugation at 2000 rpm for 15 min at 4°C, and plasma concentrations of glucose (Calbiochem) and TAG (LabAssay TAG; Wako Chemicals) were analysed using colorimetric assay kits. ELISA kits were used to determine plasma concentrations of glucagon-like peptide 1 (GLP-1; Millipore), leptin and insulin (Crystal Chem). Homeostasis model assessment of insulin resistance was calculated as described previously( Reference McAllan, Keane and Schellekens 4 ).
Tissue gene expression
Total RNA was isolated from tissues of interest (epididymal adipose tissue, liver, skeletal muscle, duodenum and ileum) using RNeasy mini kits (Qiagen) with subsequent DNase treatment to prevent genomic DNA contamination. Duodenal and ileal total RNA was isolated from representative intact tissue segments. Complementary DNA was synthesised from 1 μg of isolated total RNA and subjected to real-time quantitative PCR analysis, as described previously( Reference McAllan, Keane and Schellekens 4 ). Primer sequences of target and reference genes are listed in online Supplementary Table S2. The mRNA expression of target genes was calculated by the 2− ΔΔCp (crossing point) method, after normalising against the expression of the reference gene according to the following formula:

β-Actin (adipose, liver, muscle, duodenum and ileum), tyrosine 3-monooxygenase/tryptophan 5-monooxygenase activation protein, zeta polypeptide (YWHAZ) (liver, duodenum and ileum) and 18S (adipose) were used as the reference genes. In instances where two housekeeping genes were used, a geometric mean was determined for normalisation. Gene expression is shown relative to the LF-CAS group.
Statistical analysis
Data are presented as means with their standard errors. The effects of dietary treatments were analysed by unpaired t tests. A two-way repeated-measures ANOVA with Bonferroni's multiple comparisons was used to analyse body-weight trajectories over the treatment periods. Mann–Whitney U tests were used to analyse non-parametric data. VO2 data were analysed by ANCOVA (SAS software version 9.3), with total body weight being used as the covariant, as described previously( Reference Tschop, Speakman and Arch 10 ). Significance was set at P≤ 0·05, using GraphPad Prism (version 6; GraphPad Software Inc.), SAS software (version 9.3; SAS Institute Inc.) and Minitab (version 15; Minitab Ltd).
Results
Effect whey protein isolate on weight gain and body composition
Cumulative energy intake was significantly reduced in the LF-WPI group over the first 13 weeks, and this significance was observed from week 11 onwards (P< 0·05; Fig. 1(d)). In contrast, body-weight gain was not significantly different at week 11 (6·17 (se 0·28) g for the LF-WPI group v. 7·05 (se 0·54) g for the LF-CAS group; P= 0·17), but reached significance by week 12 (5·89 (se 0·24) g for the LF-WPI group v. 7·5 (se 0·61) g for the LF-CAS group; P< 0·05). Although after 15 weeks of dietary treatments, total body-weight gain was significantly reduced in the LF-WPI group compared with the LF-CAS group (P< 0·05; Fig. 1(b)), there was no significant difference observed in either body weight or body composition (percentage of fat or lean mass) between the two groups (Fig. 1(a) and (c), respectively). Consistent with the overall percentage of body fat mass, the mass of both subcutaneous and epididymal adipose tissue depots was not significantly different between the LF-CAS and LF-WPI groups (Fig. 2(a)).

Fig. 1 Effect of whey protein isolate on energy balance-related parameters. Mice were fed a diet containing either 10 % energy from fat and 35 % energy from sucrose with 20 % energy from casein (LF-CAS) or 10 % energy from fat and 35 % energy from sucrose with 20 % energy from whey protein isolate (LF-WPI) for 15 weeks. (a) Body-weight trajectories over the period of 15 weeks are shown (, LF-CAS;
, LF-WPI), together with (b) weight gain, (c) body composition and (d) cumulative energy intake over the first 13 weeks.
, LF-CAS;
, LF-WPI. Values are means (n 8), with their standard errors represented by vertical bars. Mean value was significantly different from that of the LF-CAS group: * P< 0·05, ** P< 0·01.
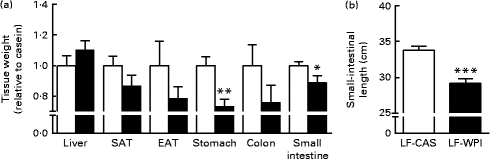
Fig. 2 Effect of whey protein isolate on (a) tissue weight and (b) small-intestinal length. Mice fed a diet containing either 10 % energy from fat and 35 % energy from sucrose with 20 % energy from casein (, LF-CAS) or 10 % energy from fat and 35 % energy from sucrose with 20 % energy from whey protein isolate (
, LF-WPI) for 15 weeks. Tissue weight is shown relative to casein. Values are means (n 8), with their standard errors represented by vertical bars. Mean value was significantly different from that of the LF-CAS group: * P< 0·05, ** P< 0·01, *** P< 0·001. SAT, subcutaneous white adipose tissue; EAT, epididymal white adipose tissue.
Effect of whey protein isolate on energy intake and metabolic parameters
A further analysis of energy intake using the TSE PhenoMaster cages in week 14 (Table 1) showed a reduction of energy intake during the dark phase in the LF-WPI group, although this was not significant. Also, no significant effects were observed for the 24 h meal pattern, represented by meal number and size, RER, and water intake; however, there was a reduction in VO2 during the dark phase in the LF-WPI group and also a trend towards a decrease in VO2 during the light phase (Table 1), as revealed by the analysis of metabolic data independent of the body weights of mice.
Table 1 Metabolic parameters in mice fed a diet containing either 10 % energy from fat and 35 % energy from sucrose with 20 % energy from casein (LF-CAS) or 10 % energy from fat and 35 % energy from sucrose with 20 % energy from whey protein isolate (LF-WPI) at week 14 (Mean values with their standard errors)

VCO2, CO2 production.
Effect of whey protein isolate on the gastrointestinal tract
The LF-WPI group had reduced stomach and small-intestinal weights relative to the LF-CAS group after 15 weeks of dietary treatments, which also corresponded with a concomitant reduction in small-intestinal length (Fig. 2).
At the cellular level, WPI intake reduced the gene expression of Wingless/int-1 5a (Wnt5a) and frizzled 4 (Fzd4) in the stomach, but not the expression of receptor tyrosine kinase-like orphan receptor 2 (Ror2) and LDL receptor-related protein 5 (Lrp5) (Fig. 3(a)). In the ileum, WPI increased the mRNA expression of Wnt5a and caused a trend towards an increase in the gene expression of Fzd4 (P= 0·094; Fig. 3(c)). In the duodenum, the Wnt signalling-associated genes did not respond to the WPI treatment (Fig. 3(b)). However, in the same region, WPI intake caused a trend towards a decrease in the gene expression of insulin receptor (IR) and Na–glucose co-transporter 1 (SGLT-1), with no change in the expression of insulin receptor substrate 1 (IRS-1), GLUT2 or glucose-dependent insulinotrophic peptide (GIP) (Table 2). WPI intake decreased the gene expression of cholecystokinin (CCK), but not significantly (Table 2). In the ileum, WPI intake increased the gene expression of GLUT2, but decreased the expression of fatty acid transporter protein 4 (FATP4) (Table 2). The expression of proglucagon in the ileum was unaffected by WPI intake (Table 2), which correlated with plasma GLP-1 levels (Table 3); however, WPI reduced the gene expression of peptide YY (PYY) (Table 2).

Fig. 3 Effect of whey protein isolate on the gene expression of gastrointestinal Wnt signalling. Mice were fed a diet containing either 10 % energy from fat and 35 % energy from sucrose with 20 % energy from casein (, LF-CAS) or 10 % energy from fat and 35 % energy from sucrose with 20 % energy from whey protein isolate (
, LF-WPI) for 15 weeks. mRNA expression levels were measured in the (a) stomach, (b) duodenum and (c) ileum. Gene expression is shown relative to the LF-CAS group, which was set at 1·00. Values are means (n 6–8), with their standard errors represented by vertical bars. Mean value was significantly different from that of the LF-CAS group: ** P< 0·01. Wnt5a, Wingless/int-1 5a; Ror2, receptor tyrosine kinase-like orphan receptor 2; Fzd4, frizzled 4; Lrp5, LDL receptor-related protein 5.
Table 2 Intestinal gene expression in mice fed a diet containing either 10 % energy from fat and 35 % energy from sucrose with 20 % energy from casein (LF-CAS) or 10 % energy from fat and 35 % energy from sucrose with 20 % energy from whey protein isolate (LF-WPI) for 15 weeks* (Mean values with their standard errors)

IR, insulin receptor; IRS-1, insulin receptor substrate 1; SGLT-1, Na–glucose co-transporter 1; CCK, cholecystokinin; GIP, glucose-dependent insulinotrophic peptide; FATP4, fatty acid transporter protein 4; PYY, peptide YY.
* Gene expression is shown relative to the LF-CAS group, which was set at 1·00.
† Values differed significantly at P< 0·05.
Table 3 Plasma parameters in mice fed a diet containing either 10 % energy from fat and 35 % energy from sucrose with 20 % energy from casein (LF-CAS) or 10 % energy from fat and 35 % energy from sucrose with 20 % energy from whey protein isolate (LF-WPI) for 15 weeks (Mean values with their standard errors)

GLP-1, glucagon-like peptide 1; HOMA-IR, homeostasis model assessment of insulin resistance.
Investigation of the same tissues in a previous published study conducted in mice fed a similar LF-CAS and LF-WPI diet for 7 weeks( Reference McAllan, Skuse and Cotter 5 ) revealed that while the stomach weight was not different between the two groups (1·06 (se 0·10) for the LF-WPI group v. 1·00 (se 0·048) for the LF-CAS group; data are shown relative to the LF-CAS group, which is set at 1·00), the intestinal weight was reduced in the LF-WPI group relative to the LF-CAS group (0·73 (se 0·026) for the LF-WPI group v. 1·00 (se 0·035) for the LF-CAS group; P< 0·001; data are shown relative to the LF-CAS group, which is set at 1·00), as was the intestinal length (33·65 (se 0·38) cm for the LF-WPI group v. 35·64 (se 0·70) cm for the LF-CAS group; P< 0·05).
Effect of whey protein isolate on plasma hormones and metabolites and lipid metabolic-related gene expression
Although WPI intake did not affect plasma leptin (Table 3) and related epididymal gene expression levels (Fig. 4), or plasma levels of TAG, insulin and glucose (Table 3), epididymal expression levels of fatty acid synthase (FASN), acetyl-CoA carboxylase (ACC) and cluster of differentiation 36 (CD36) were significantly up-regulated in the LF-WPI group compared with the LF-CAS group at week 15 (Fig. 4). Additionally, there was a trend towards an increase in the gene expression of lipoprotein lipase (P= 0·08; Fig. 4). The genes involved in insulin signalling (IR, GLUT4 and IRS-1), fatty acid catabolism (β3-adrenergic receptor (β3-AR), hormone-sensitive lipase (HSL), carnitine palmitoyltransferase 1b (CPT1b) and uncoupling protein 2 (UCP-2)) and adipogenesis (PPARγ) were unaltered by WPI intake (Fig. 4). None of the insulin signalling or lipid metabolism-related genes was altered by WPI intake in the muscle or liver (see online Supplementary Tables S3 and S4), with the exception of IRS-1, which showed a trend towards a decrease in expression in the muscle of the LF-WPI group compared with the LF-CAS group (see online Supplementary Table S3).

Fig. 4 Effect of whey protein isolate on the expression of epididymal adipose tissue genes. Mice were fed a diet containing either 10 % energy from fat and 35 % energy from sucrose with 20 % energy from casein (, LF-CAS) or 10 % energy from fat and 35 % energy from sucrose with 20 % energy from whey protein isolate (
, LF-WPI) for 15 weeks. The expression levels of epididymal adipose tissue genes were measured. Gene expression is shown relative to the LF-CAS group, which was set at 1·00. The genes investigated were insulin receptor (IR), GLUT4, insulin receptor substrate 1 (IRS-1), β3-adrenergic receptor (β3-AR), hormone-sensitive lipase (HSL), carnitine palmitoyltransferase 1b, (CPT1b), uncoupling protein 2 (UCP-2), PPARγ, lipoprotein lipase (LPL), fatty acid transporter protein 1 (FATP1), cluster of differentiation 36 (CD36), fatty acid synthase (FASN), acetyl-CoA carboxylase (ACC) and leptin. Values are means (n 8), with their standard errors represented by vertical bars. Mean value was significantly different from that of the LF-CAS group: * P< 0·05, ** P< 0·01.
Discussion
The results from the present study showed that intake of WPI for 15 weeks decreased weight gain, cumulative energy intake and dark-phase metabolic activity (VO2), which was accompanied by a reduction in stomach weight and intestinal length and weight in mice fed a low-fat diet. WPI-fed mice exhibited reduced cumulative energy intake by week 11. Dark-phase metabolic activity, measured at week 14, also decreased in the LF-WPI group compared with the LF-CAS group. The reduced body-weight gain with WPI intake could thus be a consequence of the gradual decrease in cumulative energy intake occurring before the change in the metabolic rate. Intestinal satiation/satiety-related CCK and proglucagon genes were unresponsive to the intake of WPI, as was plasma GLP-1 levels. Moreover, ileal expression of PYY, which reduces energy intake( Reference Cummings and Overduin 11 ), was significantly decreased in the LF-WPI group compared with the LF-CAS group. These data suggest that modulation of the production of these satiety and satiation hormones was not the mechanism by which WPI reduces cumulative energy intake.
The present data show that WPI intake reduces intestinal length. Given the association between the reduction in intestinal length and weight loss( Reference Brolin, LaMarca and Kenler 12 ), herein, we sought to obtain further evidence supporting a link between WPI-induced changes in intestinal cellular activity and energy balance in mice by investigating nutrient-responsive genes in the duodenum and ileum. Consistent with a reduced nutrient contact with or passage through the intestinal cells, duodenal gene expression of IR and SGLT-1, which is responsive to glucose( Reference Gorboulev, Schurmann and Vallon 13 ), showed a decreased trend in the LF-WPI group compared with the LF-CAS group. In the ileum, the cellular lipid transport protein FATP4 ( Reference Stahl, Hirsch and Gimeno 14 ) and PYY, which are mainly responsive to the availability of fat( Reference Maljaars, Peters and Masclee 15 ), were both significantly decreased by WPI intake. Although duodenal mRNA expression of GLUT2, the protein product of which is predominantly found at the basolateral membrane of enterocytes( Reference Roder, Geillinger and Zietek 16 ), was increased by WPI intake, one could argue based on other nutrient-responsive genes that this change may represent a feedback mechanism to the lower availability of nutrients in the luminal side of the intestine. The intestinal gene expression data are consistent with the changes in cumulative energy intake in mice.
To ascertain the time frame of the changes in intestinal length and weight relative to energy intake, we investigated the same tissues in an earlier published study conducted in mice fed the same LF-CAS and LF-WPI diets, but the study was terminated at week 7( Reference McAllan, Skuse and Cotter 5 ). In that study, by week 7, mice fed with WPI consumed a similar energy content to their casein-fed counterparts, and had reduced intestinal weights and lengths similar to those observed in the present study. Therefore, this suggests that the reduction in cumulative energy intake, which reached significance compared with casein-fed mice by week 11, occurred after the morphological change in the intestine. It is noteworthy that obese (ob/ob) mice have longer intestines( Reference Morton and Hanson 17 ) and that the development of high-fat diet-induced obesity in mice also increases villus length and cells per villus( Reference de Wit, Bosch-Vermeulen and de Groot 18 ). Moreover, a recent study has shown that WPI intake reduced growth-related parameters within the first week of dietary intake( Reference Tranberg, Madsen and Hansen 19 ). The data from the present study also show that WPI intake reduced stomach weight in mice. In contrast, in our previous published study conducted for 7 weeks, mice fed with WPI had an unaltered stomach weight, suggesting that the reduction in stomach weight occurred between 7 and 15 weeks of WPI intake, after the reduction in intestinal length. Together, these data suggest a potential functional relationship between WPI-induced changes in the gastrointestinal tract and energy intake.
Organ development is in part regulated by the Wnt/β-catenin pathway involving Wnt proteins, Fzd and Lrp-5/6 receptor complexes, which interact and mediate the effects through β-catenin proteins( Reference MacDonald, Tamai and He 20 ). Notably, Wnt5a has been shown to act independently of the β-catenin pathway (non-canonical signalling); however, recent data suggest that it can also inhibit and stimulate the canonical Wnt/β-catenin pathway by acting through the Ror2 receptor, which has been revealed by the generation of transgenic mice overexpressing Wnt5a ( Reference van Amerongen, Fuerer and Mizutani 21 ) and by the overexpression of Fzd4 and Lrp5 in 293 cells, where experimental manipulation allowed Wnt5a to stimulate Wnt/β-catenin signalling pathway( Reference Mikels and Nusse 22 ). Notably, mice ectopically expressing Wnt5a were stillborn and had reduced stomach and intestinal tracts( Reference van Amerongen, Fuerer and Mizutani 21 ). Interestingly, targeted deletion of the Wnt5a gene in mice also caused changes in the gastrointestinal tract( Reference Cervantes, Yamaguchi and Hebrok 23 ) similar to mice overexpressing the same gene( Reference van Amerongen, Fuerer and Mizutani 21 ). Contrasting genetic manipulations of Wnt5a resulting in similar gastrointestinal features in mice suggest a complex interplay between the non-canonical Wnt5a signalling pathway and the Wnt/β-catenin pathway. This complexity is further highlighted in the present study, as the gene expression of Wnt5a and Fzd4 decreased in the stomach of the LF-WPI group compared with the LF-CAS group, while the same genes in the ileum responded to the protein intake by increasing their expression, yet the two tissues reduced in weight. In contrast, neither gene was affected by WPI in the duodenum, with Ror2 and Lrp5 remaining unchanged throughout the gastrointestinal tract where their gene expression was investigated. Importantly, the present study demonstrates that Wnt signalling-associated genes in the gastrointestinal tract are responsive to WPI. We speculate that the regional-specific expression and actions of Wnt5a and Fzd4 and associated inhibitory or stimulatory effects on the Wnt/β-catenin signalling pathway underlie the observed changes in the gastrointestinal tract of WPI-fed mice, which, in turn, modulate energy intake and body-weight gain.
Although whey protein intake has been shown to cause an insulinotropic effect( Reference Drucker 24 , Reference Gaudel, Nongonierma and Maher 25 ), in the present study, WPI did not alter plasma levels of insulin, glucose or GLP-1, intestinal expression of GIP, or insulin signalling-associated genes in the liver, muscle or epididymal tissues. The lack of an insulinotropic effect could be due to the reduction in cumulative energy intake and associated reduced nutrient availability and/or due to the high sucrose content in the diet, which has previously been shown to cause glucose intolerance and impair GLP-1 secretion in mice( Reference Sakamoto, Seino and Fukami 26 ). Notably, there was a trend towards a decrease in the expression of IR in the duodenum in response to WPI intake. It remains to be determined whether this was related to the altered intestinal morphology because insulin action in the intestine has been shown to promote greater enterocyte turnover, which correlates with the expression of IR in the villus–crypt axis( Reference Ben Lulu, Coran and Mogilner 27 ).
In the present study, there was a mismatch in the time frame of decreased cumulative energy intake and body-weight gain as the former decreased by week 11, while body-weight gain was significantly different by week 12. Even at the end of the study, the plasma TAG level was not different between the two groups of mice, nor was the percentage of fat content in the body or specifically in the epididymal and subcutaneous fat pads. In this respect, it is noteworthy that the epididymal expression of lipogenic ACC and FASN increased in association with a concurrent increased expression of the fatty acid transporter CD36( Reference Large, Peroni and Letexier 28 ). These data suggest increased lipogenesis in the epididymal adipose tissue, which may play a role in maintaining energy homeostasis causing a mismatch in the time frame between decreased cumulative energy intake and body weight. In line with this suggestion, metabolic activity, measured at week 14, also decreased by WPI intake, presumably because of decreasing cumulative energy in mice with an altered gastrointestinal tract. In contrast, in our previous published studies, WPI at 20 % energy content in a diet with 45 % energy from fat reduced fat mass and normalised energy intake without affecting metabolic activity in mice at 8 and 21 weeks compared with the casein-fed control( Reference McAllan, Keane and Schellekens 4 , Reference McAllan, Skuse and Cotter 5 ). In the latter studies, data for metabolic activity were shown relative to body weight (ml/h per kg). In light of the suggestion that ANCOVA is a more suitable statistical method to identify the effects of the diet on metabolic activity independent of body-weight changes, we reanalysed these previously published data using ANCOVA( Reference Tschop, Speakman and Arch 10 ). Again, as with our previously published VO2 data (in ml/h per kg)( Reference McAllan, Keane and Schellekens 4 ), there was no significant effect of WPI on VO2 (ml/h) independent of the body weight in mice fed a high-fat diet with WPI (data not shown). Thus, the contrasting effects of WPI on energy intake, fat mass and metabolic activity in the previously published studies( Reference McAllan, Keane and Schellekens 4 , Reference McAllan, Skuse and Cotter 5 ) compared with the present study could be related to how whey proteins affect energy balance in a background of altered fat to carbohydrate content in the diet.
In conclusion, the results herein show that 20 % energy from WPI in a diet containing 10 % energy from fat and 35 % energy from sucrose reduced cumulative energy intake, dark-phase metabolic activity and body-weight gain. This is accompanied by a decreased intestinal length and weight, as well as stomach weight, which was correlated with an altered expression of Wnt signalling-associated genes. Further investigations are required to determine the significance of the alteration to the intestinal environment uncovered herein for the regulation of energy balance.
Supplementary material
To view supplementary material for this article, please visit http://dx.doi.org/10.1017/S0007114514004024
Acknowledgements
K. N. N. was supported by the Teagasc Vision Programme on Obesity (RMIS5974). L. M. was supported by the Teagasc Walsh Fellowship. J. R. S. was supported by a 1000-talents professorship from the Chinese government. The funding bodies had no input on the design of the study or in the interpretation of the data.
The authors' contributions are as follows: L. M., J. R. S., J. F. C. and K. N. N. designed the study; K. N. N. and J. F. C. obtained ethical approval for the study; L. M. performed the experiments; L. M. and J. R. S. analysed the data; L. M. generated the figures. All authors contributed to the drafting of the manuscript. All authors approved the final version for submission.
The authors declare that there is no competing interest.