Introduction: the history of HMOs
Human breast milk is considered the gold standard nutrient source for infants in the early stages of life due to the presence of several remarkable functional ingredients (Van den Abbeele et al., Reference Van den Abbeele, Duysburgh, Vazquez, Chow, Buck and Marzorati2019). One group of such ingredients is the human milk oligosaccharides (HMOs). A classification which is given to a group of structurally diverse and complex unconjugated glycans present in human breast milk (Ninonuevo et al., Reference Ninonuevo, Park, Yin, Zhang, Ward, Clowers, German, Freeman, Killeen, Grimm and Lebrilla2006). HMOs were first “discovered” towards the end of the nineteenth century after French biochemist Georges Denigés noted that in addition to lactose, human and bovine milk possessed several other carbohydrate structures with Polonowski and Lespagnol originally terming these unknown fractions as gynolactoses (Polonowski and Lespagnol, Reference Polonowski and Lespagnol1929, Reference Polonowski and Lespagnol1931). The levels of HMOs present in breast milk range from 5 to 25 g/L throughout the course of lactation, making the levels of oligosaccharides present in human milk the highest amongst mammalian species. This is over 100 times greater than the oligosaccharide content found in bovine milk, which has been estimated at around 100 mg/L (Robinson, Reference Robinson2019; Zivkovic et al., Reference Zivkovic, German, Lebrilla and Mills2011).
Structural complexity of HMOs
Virtually, all HMOs possess a lactose (Lac) core to which a multitude of different monosaccharide “building blocks,” including galactose (Gal), glucose (Glc), fucose (Fuc), sialic acid (Neu5Ac) and N-acetylglucosamine (GlcNAc), can be attached via the action of specific glycosyltransferases in the presence of α-lactalbumin (Smilowitz et al., Reference Smilowitz, Lebrilla, Mills, German and Freeman2014). The synthesis of HMOs begins with the enzymatic elongation of lactose (Lac) by either β1-3 or β1-6 linkages of Gal to lacto-N-biose (LNB) or N-acetyllactosamine (LacNAc), respectively. Based on this, HMOs can be classified as either Type-I or Type-II chains. Type-I chain HMOs possess lacto-N-tetraose (LNT), which is lactose coupled to LNB. While Type-II chains, are composed LNT isomer lacto-N-neotetraose (LNnT), and is Lac linked to LacNAc (James et al., Reference James, Motherway, Bottacini and van Sinderen2016). These core HMO structures can then be further elongated and additionally categorised as neutral, fucosylated or sialylated (Plaza-Diaz et al., Reference Plaza-Diaz, Fontana and Gil2018; Zivkovic et al., Reference Zivkovic, German, Lebrilla and Mills2011). Neutral HMOs possess structures similar to galacto-oligosaccharides (GOSs) containing both Glc and Gal (Barile and Rastall, Reference Barile and Rastall2013), and may also accommodate several GlcNAc or LNB units attached via β1-3 and β1-6 linkages (Ayechu-Muruzabal et al., Reference Ayechu-Muruzabal, van Stigt, Mank, Willemsen, Stahl, Garssen and van’t Land2018). At this point, Fuc units can be enzymatically attached via α1-2, α1-3 or α1-4 linkages generating fucosylated HMOs. Hereafter, one or more molecules of Neu5Ac may be attached via α2-3 or α2-6 linkages in the presence of sialyl-transferases generating sialylated HMOs (Smilowitz et al., Reference Smilowitz, Lebrilla, Mills, German and Freeman2014). Figure 1 gives a generalised overview of the complexity and structural diversity of HMOs present in breast milk.
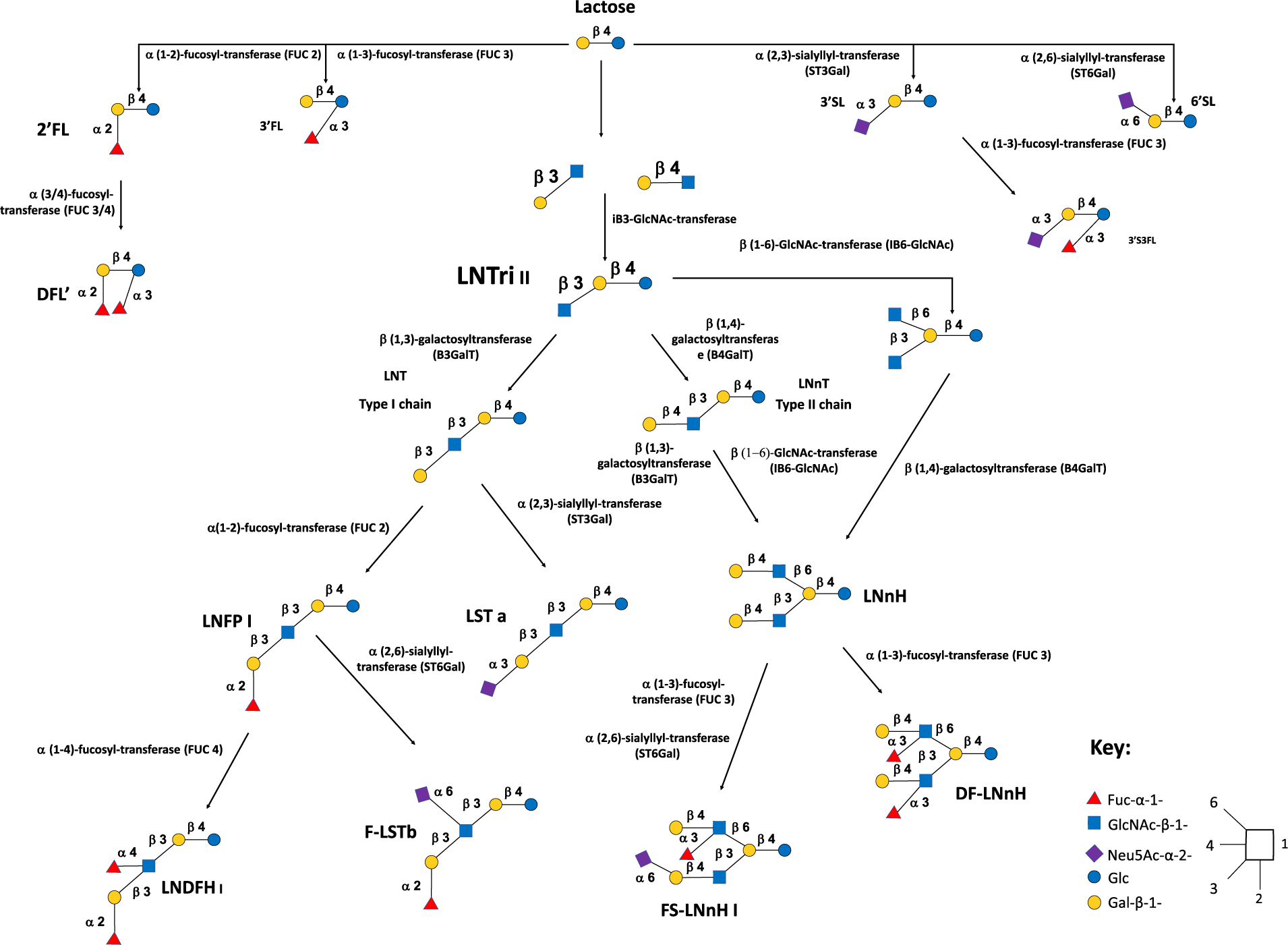
Figure 1 Generalised overview of the complexity and structural diversity of human milk oligosaccharides (HMOs) present in breast milk.
Abbreviations: Neutral HMOs: 2’FL, 2′-fucosyllactose; 3’FL, 3′-fucosyllactose; DFL, difucosyllactose; DF-LNnH, difucosylated lacto-N-neohexaose; LNDFH I, lacto-N-difucohexaitol I; LNFP I, lacto-N-fucopentaose I; LNnH, lacto-N-neohexaose; LNnT, lacto-N-neotetraose; LNT, lacto-N-tetraose. Acidic non-fucosylated HMOs: 3’SL, 3′-sialyllactose; 6’SL, 6′-sialyllactose; LST a, sialyllacto-N-tetraose a. Acidic fucosylated HMOs: 3’S3FL, 3’-Sialyl-3-fucosyllactose; F-LST b, sialylfucosyllacto-N-tetarose b; FS-LNH I, fucosylsiallacto-N-hexose I.
Composition of human milk oligosaccharides in breast milk and factors affecting composition
To date, somewhere in the region of 200 HMOs have been identified in the breast milk of mothers with the most widely recognised HMOs being 2′-fucosyllactose (2’FL), LNT and LNnT (Barile and Rastall, Reference Barile and Rastall2013; Egge et al., Reference Egge, Dell and Von Nicolai1983; Urashima et al., Reference Urashima, Hirabayashi, Sato and Kobata2018). The composition and concentration of HMOs present in breast milk are highly dependent on several critical factors, including geographical location, ethnicity, length of gestation and secretor status. In general, the levels of HMOs present in breast milk are highest immediately following birth and decrease throughout lactation with the concentration of 2′FL appearing to be highest during the first month postpartum (Thurl et al., Reference Thurl, Munzert, Henker, Boehm, Muller-Werner, Jelinek and Stahl2010, Reference Thurl, Munzert, Boehm, Matthews and Stahl2017; Xu et al., Reference Xu, Davis, Goonatilleke, Smilowitz, German and Lebrilla2017). Yet, the rates at which HMOs decline are not constant across all HMOs. For example, while 2’FL, difucosyllactose (DFL), lacto-N-fucopentaose-2 (LNFP II), 3’-Sialyllactose (3’SL) and 6’-Sialyllactose (6′SL) all decline in concentration throughout lactation, the rate of decline does not appear to significantly alter during days 30–120 (Gabrielli et al., Reference Gabrielli, Zampini, Galeazzi, Padella, Santoro, Peila, Giuliani, Bertino, Fabris and Coppa2011; Spevacek et al., Reference Spevacek, Smilowitz, Chin, Underwood, German and Slupsky2015; Thurl et al., Reference Thurl, Munzert, Henker, Boehm, Muller-Werner, Jelinek and Stahl2010), whereas lacto-N-fucopentaose-1 (LNFP I) appears to decline just 3 days after birth recording a twofold decline by the end of lactation, respectively (Bao et al., Reference Bao, Chen and Newburg2013; Smilowitz et al., Reference Smilowitz, O’Sullivan, Barile, German, Lonnerdal and Supsky2013). Other HMOs, including 3′-fucosyllactose (3’FL), appear to increase in concentration throughout lactation by 1.67–1.8-fold (Austin et al., Reference Austin, De Castro, Sprenger, Binia, Affolter, Garcia-Rodenas, Beauport, Tolsa and Fumeaux2019; Gabrielli et al., Reference Gabrielli, Zampini, Galeazzi, Padella, Santoro, Peila, Giuliani, Bertino, Fabris and Coppa2011; Samuel et al., Reference Samuel, Binia, de Castro, Thakkar, Billeaud, Agosti, Al-Jashi, Costeire, Marchinr, Martinez-Costa, Picaud, Stiris, Stoicescu, Vanpee, Domellof, Austin and Sprenger2019; Smilowitz et al., Reference Smilowitz, O’Sullivan, Barile, German, Lonnerdal and Supsky2013).
However, a significant percentage of mothers can only synthesise certain HMOs, including 2’FL, dependant on their secretor status. Secretor status is based on the Lewis blood group and depends on the expression of the specific glycosyltransferases, α1-2-fucosyltransferase (FUT2 encoded by the Se gene) and α1-3/4-fucosyltransferase (FUT3 encoded by the Le gene), and can result in marked differences in which HMOs are synthesised (Blank et al., Reference Blank, Dotz, Geyer and Kunz2012; Hegar et al., Reference Hegar, Wibowo, Basrowi, Ranuh, Sudarmo, Munasir, Atthiyah, Widodo, Supriatmo, Kadim, Suryawan, Diana, Manoppo and Vandenplas2019). Table 1 summarises these findings.
Table 1. Human milk oligosaccharide composition of breast milk based on the genetic background of the mother. Source: Vandenplas et al. (Reference Vandenplas, Berger, Carnielli, Ksiazyk, Lagstrom, Luna, Migacheva, Mosselmans, Picaud, Possner, Singhal and Wabitsch2018).

However, the Lewis antigen system and secretor status can be described as an over generalisation of an extremely complex situation (Plaza-Diaz et al., Reference Plaza-Diaz, Fontana and Gil2018) as even when these factors are accounted for, substantial differences in HMO profile can still occur. For example, FUT2 and FUT3 have been shown to compete for several of the same substrates. As a result, differences in levels of expression can alter the synthesis of 2’FL by a “secretor” mother (McGuire et al., Reference McGuire, Meehan, McGuire, Williams, Foster, Sellen, Kamau-Mbuthia, Kamundia, Mbugua, Moore, Prentice, Kvist, Otoo, Brooker, Price, Shafii, Placek, Lackey, Robertson, Manzano, Ruiz, Rodriguez, Pareja and Bode2017; Plaza-Diaz et al., Reference Plaza-Diaz, Fontana and Gil2018).
Additionally, levels and composition of HMOs present in breast milk may also vary between the mothers of preterm and full-term infants regardless of secretor status. However, reports on this are contradictory with several papers seemingly reporting that HMOs may or may not vary between preterm and term infant mothers (Austin et al., Reference Austin, De Castro, Sprenger, Binia, Affolter, Garcia-Rodenas, Beauport, Tolsa and Fumeaux2019; De Leoz et al., Reference De Leoz, Gaerlan, Strum, Dimapasoc, Mirmiran, Tancredi, Smilowitz, Kalanetra, Mills, German, Lebrilla and Underwood2012; Kunz and Rudloff, Reference Kunz and Rudloff2017; Thurl et al., Reference Thurl, Munzert, Boehm, Matthews and Stahl2017).
Furthermore, the sizeable heterogeneity in the levels and composition of HMOs detected in breast milk may be in part due to differences in analytical techniques used in studies, including high-performance liquid chromatography mass spectrometry (HPLCMS) and HPLC time-of-flight mass spectrometry, high-performance anion-exchange chromatography with pulsed amperometric detection (HPAEC-PAD), as well as differences in the number of preparation steps taken (van Leeuwen, Reference van Leeuwen2019; Zivkovic et al., Reference Zivkovic, German, Lebrilla and Mills2011). A further complication is the lack of analytical standards needed in order to quantitatively assess the concentration of HMOs present (Wicinski et al., Reference Wicinski, Sawicka, Gebalski, Kubiak and Malinowski2020). As a result, it could be argued that determining the average composition and quantity of HMOs in breast milk, based on current published information, is not achievable due to too many confounding variables. Furthermore, to reiterate (Thurl et al., Reference Thurl, Munzert, Boehm, Matthews and Stahl2017), to be able to determine the average composition of HMOs in breast milk, a worldwide multicentre study following the same protocols would be required. However, even if the average HMO content of breast milk was determined, what relevance this would have regarding clinical significance remains uncertain.
Human milk oligosaccharides, health benefits and clinical data
It is well documented that breastfeeding is highly associated with several health benefits, including improved growth rate, lower prevalence of respiratory, intestinal and urinary infections (Li et al., Reference Li, Dee, Li, Hoffman and Grummer-Strawn2014) and lower incidence of allergies and autoimmune conditions, with some of this being put down to the presence of HMOs in breast milk (Doherty et al., Reference Doherty, Lodge, Dharmage, Dai, Bode and Lowe2018; Triantis et al., Reference Triantis, Bode and van Neerven2018).
The structural nature of HMOs renders them resistant to digestive enzymes found within the GI tract (Garrido et al., Reference Garrido, Barile and Mills2012a) with >90 per cent of HMOs reaching the colon intact. HMOs likely function as prebiotics, stimulating the growth of beneficial bacteria, including bifidobacteria (Barile and Rastall, Reference Barile and Rastall2013; Bode, Reference Bode2009). HMOs can also act as soluble decoys, preventing the adhesion of pathogenic bacteria to cell surface receptors due to their resemblance to the glycans found on the surface of epithelial cells in the intestinal tract (Wicinski et al., Reference Wicinski, Sawicka, Gebalski, Kubiak and Malinowski2020). In addition, the formation of the short-chain fatty acids (SCFAs) butyrate, acetate and propionate from saccharolytic fermentation plays vital roles in the activation and differentiation of immune cells and may also reduce the risk of infections and allergies (Ayechu-Muruzabal et al., Reference Ayechu-Muruzabal, van Stigt, Mank, Willemsen, Stahl, Garssen and van’t Land2018; Kumari and Kozyrskyj, Reference Kumari and Kozyrskyj2017). Additionally, it has been shown in infants that a lack of bifidobacteria, particularly those associated with HMO degradation and utilisation, is associated with increases in systemic inflammation and immune dysfunction (Henrick et al., Reference Henrick, Rodriguez, Lakshmikanth, Pou, Henckel, Arzoomand, Olin, Wang, Mikes, Tan, Chen, Ehrlich, Bernhardsson, Mugabo, Ambrosiani, Gustafsson, Chew, Brown, Prambs and Brodin2021). Furthermore, supplementation of 2’FL in combination with B. pseudocatenulatum MP80 was associated with changes in gene expression of both anti-inflammatory and pro-inflammatory markers in the cecum of healthy mice, whereas in mice with dextran sulphate sodium (DSS)-induced colitis, supplementation of 2’FL in combination with B. pseudocatenulatum MP80 resulted in attenuations of bodyweight loss, along with a reduction in DSS-induced immune cell infiltration, increase in colon length and disrupted mucosal architecture along with preventing a reduction in occludin expression (Heiss et al., Reference Heiss, Ehrlich, Maldonado-Gomez, Taft, Larke, Goodson, Slupsky, Tancredi, Raybould and Mills2021).
However, not all infants are breastfed, with many being fed formula milk which may or may not be supplemented with HMOs. The first two HMOs to be commercially produced were 2’FL and LNnT, and while these compounds are referred to as HMOs, they are not sourced from human milk, but are produced by microbial fermentation using strains of E. coli and yeasts which have been genetically modified (Sprenger et al., Reference Sprenger, Lee, De Castro, Steenhout and Thakkar2017).
Several studies have suggested that infants fed HMO-supplemented formula milk present lower risks of parent-reported bronchitis and respiratory infections, along with reduced use of antibiotics, less waking at nights and improved age-appropriate growth (Marriage et al., Reference Marriage, Buck, Goehring, Oliver and Williams2015; Puccio et al., Reference Puccio, Alliet, Cajozzo, Janssens, Corsello, Sprenger, Wernimont, Egli, Gosoniu and Steenhout2017). In a study on the 2’FL, solely or in combination with Bifidobacterium longum subsp. infantis (Bi-26), on cognitive and structural development in young pigs (Sutkus et al., Reference Sutkus, Joung, Hirvonen, Jensen, Ouwehand, Mukherjea, Donovan and Dilger2022), the authors noted that synbiotic administration of 2’FL and Bi-26 had several interactive effects on microstructural brain components; however, it appeared to have no effect on memory. Preclinical in vitro and in vivo studies propose that both 2′FL and LNnT promote the growth of several Bifidobacterium and Bacteroides species, strains, and subspecies including B. longum subsp. infantis, Bacteroides fragilis, and Bacteroides vulgatus, amongst others (Marcobal et al., Reference Marcobal, Barboza, Froehlich, Block, German, Lebrilla and Mills2010; Yu et al., Reference Yu, Chen and Newburg2013).
However, while the importance of breast milk on infant health outcomes is well supported, there remains a great deal unknown regarding the efficacy of HMOs added to infant formulas on infant health outcomes. Furthermore, it has been demonstrated that several species, strains and subspecies of microorganisms found within the gut microbiota, including Bifidobacterium adolescentis and Bifidobacterium animalis, do not grow well on HMOs, including 2′FL and LNnT on their own (Lawson et al., Reference Lawson, O’Neill, Kujawska, Javavdi, Wijeyesekera, Flegg, Chalklen and Hall2020; LoCascio et al., Reference LoCascio, Desai, Sela, Weimer and Mills2010; Marcobal et al., Reference Marcobal, Barboza, Froehlich, Block, German, Lebrilla and Mills2010; Sela et al., Reference Sela, Garrido, Lerno, Wu, Tan, Eom, Joachimiak, Lebrilla and Mills2012; Xiao et al., Reference Xiao, Takahashi, Nishimoto, Odamaki, Yaeshima, Iwatsuki and Kitaoka2010). This suggests that the composition of gut microbiota may be key if formula milks containing 2’FL and LNnT are to be effectively utilised. Therefore, the rest of this review will focus on the effects that HMOs have on the composition of the infant gut microbiota both in vitro and in vivo and will attempt to determine the metabolic fate of HMOs.
The metabolic fate of human milk oligosaccharides: infant and rodent studies
It was established as early as the 1970s that HMOs are present in the urine of expecting mothers as soon as 8 weeks (Hallgren et al., Reference Hallgren, Lindberg and Lundblad1977) and can be detected in the mammary glands using 13C isotopes (Dotz et al., Reference Dotz, Rudloff, Blank, Lochnit, Geyer and Kunz2014), suggesting that HMOs circulate throughout maternal serum. Hirschmugl et al. (Reference Hirschmugl, Brandl, Csapo, van Poppel, Kofeler, Desoye, Wadsack and Jantscher-Krenn2019) investigated individual and temporal variations in the composition and levels of HMOs in maternal serum throughout the course of pregnancy. In this study, serum samples were collected from healthy pregnant woman throughout the course of gestation at weeks 10–14 (Visit 1), 20–24 (Visit 2) and 30–35 (Visit 3) and at the time of admission to delivery at the Department of Obstetrics, Medical University of Graz, Graz, Austria. In total, 16 HMOs [2′FL, 3’FL, DF’, 3′SL, 6′SL, LNT, LNnT, LNFP I, II, and III, LST a, b, and c, LNDFH, LNH and disialyllacto-N-tetraose (DSLNT)] were detected in serum with the authors reporting a steady increase in the presence of HMOs, in particular, fucosylated HMOs in circulation throughout the course of pregnancy.
Based on this, one could speculate that HMOs may undergo maternal-to-foetal transport. Indeed, there are data suggesting that several HMOs, including 2′FL, 3’FL, DFL and 6′SL, were present in amniotic fluid (Wise et al., Reference Wise, Robertson, Choudhury, Rautava, Isolauri, Salminen and Bode2018). Likewise, it has also been demonstrated, using an ex vivo experimental model involving isolated placental cotyledons perfused with 2’FL, that 2’FL was able to cross the placenta (Hirschmugl et al., Reference Hirschmugl, Brandl, Csapo, van Poppel, Kofeler, Desoye, Wadsack and Jantscher-Krenn2019). Furthermore, given that HMOs function as signalling molecules and that similar glycan structures can act as receptors in cells and tissues (Bhargava et al., Reference Bhargava, Li, Stanya, Jacobi, Dai, Liu, Gangl, Harn and Lee2012), it is likely that HMOs may also contribute towards the development and functioning of immune (Plaza-Diaz et al., Reference Plaza-Diaz, Fontana and Gil2018) and endothelial cells (Donovan and Comstock, Reference Donovan and Comstock2016) of infants in utero. However, since foetal circulation and cord blood are not accessible during pregnancy, these findings should be interpreted with a great deal of care (Hornef and Penders, Reference Hornef and Penders2017; Walker et al., Reference Walker, Clemente, Peter and Loos2017).
To date, the ability of HMOs to end up in the urine of infants has been extensively studied using 13C-labelled glycans along with several analytical techniques, including MALDI-TOF-MS, HPAEC-PAD, nano-LC–MS and MALDI FT-ICR MS. The results of these studies suggest that fully and partially intact HMOs, including 2’FL, 3’SL, 6’SL, LNT and LNnT, can be present in the urine of infants, albeit in far lower concentrations than that found in milk at just 4 per cent, with secretor/non-secretor status massively impacting on which HMOs are detected (Borewicz et al., Reference Borewicz, Gu, Saccenti, Hechler, Beijers, de Weerth, van Leeuwen, Schols and Smidt2020; De Leoz et al., Reference De Leoz, Wu, Strum, Ninonuevo, Gaerlan, Mirmiran, German, Mills, Lebrilla and Underwood2013; Dotz et al., Reference Dotz, Rudloff, Blank, Lochnit, Geyer and Kunz2014, Reference Dotz, Rudloff, Meyer, Lochnit and Kunz2015; Goehring et al., Reference Goehring, Kennedy, Prieto and Buck2014).
Given that HMOs can end up in the urine of infants, HMOs can clearly be absorbed through the epithelial cells of the gastrointestinal tract and enter the bloodstream (Dotz et al., Reference Dotz, Rudloff, Blank, Lochnit, Geyer and Kunz2014; Rudloff et al., Reference Rudloff and Kunz2012). To date, the potential for HMOs to enter circulation has been extensively studied in animal models. In one study, conducted in rats fed both mixed and isolated HMOs, including 2’FL, it was noted that when mixed HMOs were ingested, 2’FL was detected in circulation 30 min later in a dose-dependent manner, reaching a maximum at 60 min (Vazquez et al., Reference Vazquez, Santos-Fandila, Buck, Rueda and Ramirez2017), whereas in rats fed isolated HMOs, levels of 2’FL detected in circulation similarly increased in concentration over time, again in a dose-dependent manner. However, they did not reach a maximum peak within the 4-h sampling period. In contrast, Jantscher-Krenn et al. (Reference Jantscher-Krenn, Marx and Bode2013) reported that only 3’SL, along with very few other HMOs, was detected in the serum of rats. The discrepancies in findings between these studies probably result from biological differences in the metabolism of HMOs due to the ages of rats used in each respective study.
The potential for HMOs to enter into the circulation and plasma of infants was investigated by Ruhaak et al. (Reference Ruhaak, Stroble, Underwood and Lebrilla2014) in 13 full-term infants using solid-phase extraction followed by an analysis by nHPLC-PGC-chip-TOF-MS. In total, 15 oligosaccharides, including LNT, LDFP, LNFT, 3’SL, 6’SL, 3′sialyllactosamine (3’SLN), 6′sialyllactosamine (6’SLN), LNFP III and 2’FL, were detected in the plasma of infants, albeit in lower concentrations than levels found in breast and formula milk, with over a 10-fold variation in LNT being recorded between partially breastfed and formula-fed infants. Interestingly, an unknown isomer of SLN was found. Given SLN is usually derived from bovine milk (McGrath et al., Reference McGrath, Fox, McSweeney and Kelly2016; Ruhaak et al., Reference Ruhaak, Stroble, Underwood and Lebrilla2014) and the relative abundances detected in the infants of this study far exceed those found in bovine milk (Fong et al., Reference Fong, Ma and McJarrow2011). From this, one could theorise that new SLNs detected in the circulation of infants may have been produced via interactions between milk, milk by-products and bacterial glycosidases (Lis-Kuberka and Orczyk-Pawilowicz, Reference Lis-Kuberka and Orczyk-Pawilowicz2019).
Moreover, using isotopically labelled standards, coupled with ultra-performance liquid chromatography and HPLC, Goehring et al. (Reference Goehring, Kennedy, Prieto and Buck2014) investigated the presence of HMOs in both breastfed and formula-fed infants. The authors reported the detection of several HMOs, including 2′FL, 3’FL and LNnT, in the plasma of breastfed but not formula-fed infants, again albeit in far lower levels. A similar finding was reported by Radzanowski et al. (Reference Radzanowski, Garrett, Li and Anita2013), who documented that the concentration of HMOs present in infant plasma was substantially less (3′SL: 0.10–0.78 mg/L; 6′SL: 0.05–0.68 mg/L; 2′FL: 0–2.25 g/L) compared with breast milk (3′SL: 54.3–225 mg/L; 6′SL: 29.3–726 mg/L; 2′FL: 0–3.8 g/L) at just 0.1 per cent, respectively.
The degradation and transportation of HMOs
As previously discussed, virtually, all HMOs possess a lactose core to which a multitude of different monosaccharide “building blocks,” including Gal, Glc, Fuc, sialic acid (Neu5Ac) and N-acetylglucosamine (GlcNAc), can be attached via the action of specific glycosyltransferases in the presence of α-lactalbumin (Smilowitz et al., Reference Smilowitz, Lebrilla, Mills, German and Freeman2014). In order to stimulate the fructose 6-phosphate phosphoketolase-dependent glycolytic pathway more commonly termed the bifid shunt active in bifidobacteria, these complex milk glycans must be degraded (Pokusaeva et al., Reference Pokusaeva, Fitzgerald and van Sinderen2011). The mechanisms by which HMOs undergo degradation can be characterised as intracellular (transport-dependant) and extracellular (glycosidase-dependent). Both mechanisms require the use of specific ATP (adenosine triphosphate)-binding cassette (ABC) transporters to either import intact or processed glycans inside the bacterial cell (Garrido et al., Reference Garrido, Kim, German, Raybould and Mills2011) with the most common strains of bifidobacteria (B. breve, B. longum and Bifidobacterium bifidum) preferring to utilise specific mechanisms of action (Sakanaka et al., Reference Sakanaka, Gotoh, Yoshida, Odamaki, Koguchi, Xiao, Kitaoka and Katayama2020). See Figure 2.
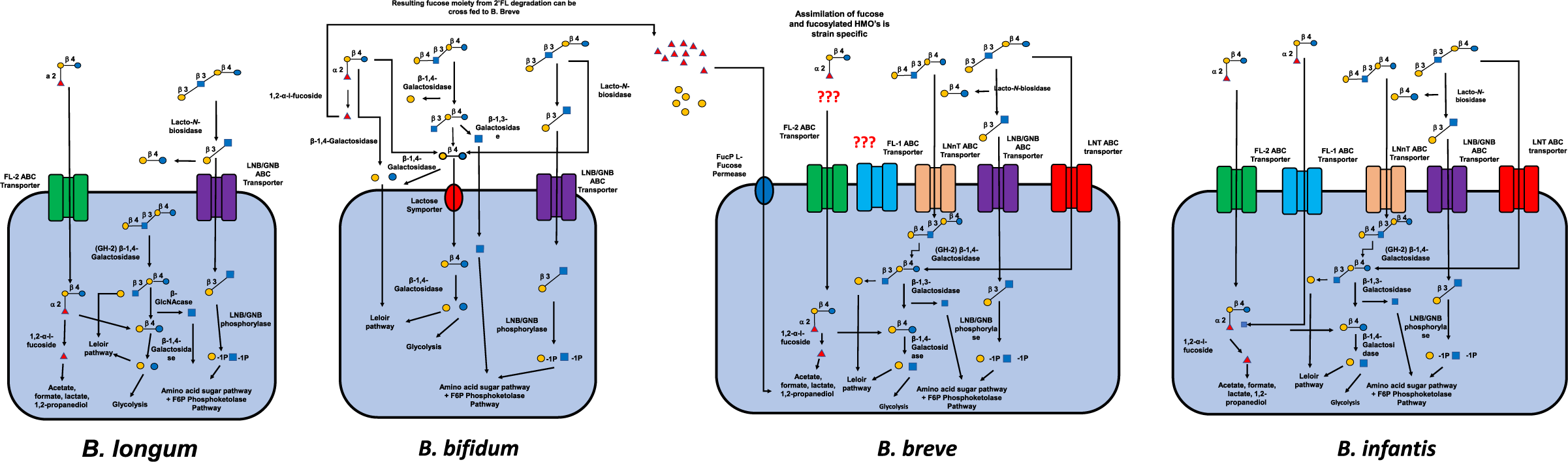
Figure 2 Intracellular and extracellular degradation of the three main human milk oligosaccharides (2’FL, LNT and LNnT) and resulting metabolites by four common species of Bifidobacterium (Bifidobacterium longum, B. bifidium, B. breve and B. longum) and selective fermentation pathways. Abbreviations: ABC, ATP-binding cassette; FL, fucosyllactose; GNB, galacto-N-biose; LNB, lacto-N-biose; LNnT, lacto-N-neotetraos.
Fucosidases and fucosylated human milk oligosaccharide transporters
To hydrolyse fucosidic-linked HMOs, two distinct glycoside hydrolase (GH) families, GH95 and GH29, are required for the degradation of specific fucosidic linkages (Sakanaka et al., Reference Sakanaka, Hansen, Gotoh, Katoh, Yoshida, Odamaki, Yachi, Sugiyama, Kurihara, Hirose, Urashima, Xiao, Kitaoka, Fukiya, Yokota, Lo Leggio, Abou Hachem and Katayama2019). While both GH95 and GH29 can target the 1,2-, 1,3- and 1-4-α-l-fucosides, GH29 displays a higher affinity towards both 1,3- and 1,4-α-l-fucosides, whereas GH95 displays a higher preference towards the hydrolysis of 1,2-α-l-fucosides (Matsuki et al., Reference Matsuki, Yahagi, Mori, Matsumoto, Hara, Tajima, Ogawa, Kodama, Yamamoto, Yamada, Matsumoto and Kurokawa2016; Shani et al., Reference Shani, Hoeflinger, Heiss, Masarweh, Larke, Jensen, Wickramasinghe, Davis, Goonatilleke, El-Hawiet, Nguyen, Klassen, Slupsky, Lebrilla and Mills2022; Zeuner et al., Reference Zeuner, Muschiol, Holck, Lezyk, Gedde, Jers, Mikkelsen and Meyer2018). The transporters responsible for the uptake of fucosyllactose (FL’) were first discovered in B. longum subsp. infantis ATCC 15697T (Sela et al., Reference Sela, Chapman, Adeuya, Kim, Chen, Whitehead, Lapidus, Rokhsar, Lebrilla, German, Price, Richardson and Mills2008). B. longum subsp. infantis ATCC 15697T possesses two paralogous FL’ transporters which share up to 60 per cent of the same solute-binding proteins (SBPs), suggesting that there is some degree of overlap in their ability to transport various HMOs (Sakanaka et al., Reference Sakanaka, Gotoh, Yoshida, Odamaki, Koguchi, Xiao, Kitaoka and Katayama2020). This overlap was demonstrated in a recent study conducted by Sakanaka et al. (Reference Sakanaka, Hansen, Gotoh, Katoh, Yoshida, Odamaki, Yachi, Sugiyama, Kurihara, Hirose, Urashima, Xiao, Kitaoka, Fukiya, Yokota, Lo Leggio, Abou Hachem and Katayama2019) with the authors concluding that FL transporter-1 was only able to import low molecular weight HMOs, including 2′FL and 3’FL. In contrast, FL transporter-2 was able to import not only 2′FL and 3’FL, but also LDFT and LNFP I. Yet, the ability of different species and strains of bifidobacteria to express FL’ transporters is not identical. As research by Garrido et al. (Reference Garrido, Ruiz-Moyano, Kirmiz, Davis, Totten, Lemay, Ugalde, German, Lebrilla and Mills2016) and Matsuki et al. (Reference Matsuki, Yahagi, Mori, Matsumoto, Hara, Tajima, Ogawa, Kodama, Yamamoto, Yamada, Matsumoto and Kurokawa2016) revealed, there was a remarkable difference in the ability of B. longum subsp. infantis and B. bifidum to utilise FL’ due to the presence of different intracellular and extracellular ABC-type transporters (K02025 and K02026).
Outside of Bifidobacterium spp., GH29 and GH95 have also been detected in Roseburia inulinivorans and GH29 detected in Akkermansia muciniphila, enabling it to cleave the α1-2-fucosyl linkage to Gal (Kostopoulos et al., Reference Kostopoulos, Elzinga, Ottman, Klievink, Blijenberg, Aalvink, Boeren, Mank, Knol, de Vos and Belzer2020).
Lacto-N-biosidase and LNB transporter
LNT is one of the most abundant neutral HMOs present in breast milk and is hydrolysed by lacto-N-biosidase (lnb), resulting in the formation of LNB and Lac (Yamada et al., Reference Yamada, Gotoh, Sakanaka, Hattie, Stubbs, Katayama-Ikegami, Hirose, Kurihara, Arakawa, Kitaoka, Okuda, Katayama and Fushinobu2017). The specificity of lnb present in Bifidobacterium also varies remarkably between species (Sakanaka et al., Reference Sakanaka, Gotoh, Yoshida, Odamaki, Koguchi, Xiao, Kitaoka and Katayama2020). On this basis, lnb present in B. bifidum is classified as GH20, whereas in B. longum, lnb is categorised as GH136. The differences being that GH136 requires an additional chaperonin for proper protein folding and is also capable of accepting sialyllacto-N-tetraose a (LST a) in addition to LNT (Sakurama et al., Reference Sakurama, Kiyohara, Wada, Honda, Yamaguchi, Fukiya, Yokota, Ashida, Kumagai, Kitaoka, Yamamoto and Katayama2013; Yamada et al., Reference Yamada, Gotoh, Sakanaka, Hattie, Stubbs, Katayama-Ikegami, Hirose, Kurihara, Arakawa, Kitaoka, Okuda, Katayama and Fushinobu2017).
In B. bifidum, degradation of HMOs to LNB and monosaccharides begins extracellularly with the hydrolysis of LNFP I, II and lacto-N-difucohexaose I, II (LNDFH I, II) to LNT and Fuc with the aid of additional fucosidases (Marcobal and Sonnenburg, Reference Marcobal and Sonnenburg2012). LNB is then hydrolysed from LNT by Lnb and then transported inside of the cell leaving any remaining fucosyl residues behind. Lnb phosphorylase then converts LNB into its respective monosaccharides Gal and GlcNAc, which then undergo further assimilation (Zivkovic et al., Reference Zivkovic, German, Lebrilla and Mills2011). In B. longum, the degradation of LNT seemingly follows similar principles to that of B. bifidum with LNB being extracellularly hydrolysed from LNT. However, unlike B. bifidum, the hydrolysis of LNB from LNFP I by B. longum occurs intracellularly, meaning that no Fuc residues are left behind (Xiao et al., Reference Xiao, Takahashi, Nishimoto, Odamaki, Yaeshima, Iwatsuki and Kitaoka2010; Yamada et al., Reference Yamada, Gotoh, Sakanaka, Hattie, Stubbs, Katayama-Ikegami, Hirose, Kurihara, Arakawa, Kitaoka, Okuda, Katayama and Fushinobu2017). In contrast to both B. bifidum and B. longum, the degradation of LNT and LNnT to LNB by B. longum subsp. infantis occurs entirely intracellularly with LNB constituent monosaccharides Gal and GlcNAc entering selective fermentation pathways (Ozcan and Sela, Reference Özcan and Sela2018). More recently, the presence of GH136 has been discovered in Roseburia with the degradation of fuscoylated pentose and hexose HMOs said to occur extracellularly (Pichler et al., Reference Pichler, Yamada, Shuoker, Alvarez-Silva, Gotoh, Leth, Schoof, Katoh, Sakanaka, Katayama, Jin, Karlsson, Arumugam, Fushinobu and Abou Hachem2020).
Sialidase
For microorganisms to be able to utilise sialic acids, they must possess the necessary sialidases required to hydrolyse the α-2,3 and α-2,6 linkages of sialylated HMOs (Kiyohara et al., Reference Kiyohara, Tanigawa, Chaiwangsri, Katayama, Ashida and Yamamoto2011; Zivkovic et al., Reference Zivkovic, German, Lebrilla and Mills2011). In bifidobacteria, the most common sialidases are classified as GH33 with each member of the GH33 family exhibiting preference for specific sialic acid linkages. For example, in B. bifidum, the sialidase present is categorised as SiaBb2 and exhibits a preference for α-2,3 linkages, whereas the sialidase present in B. longum subsp. infantis is classified as NanH2, but unlike SiaBb2 appears to exhibit an equal preference for both α-2,3 and α-2,6 linkages (Juge et al., Reference Juge, Tailford and Owen2016).
LNT β-1,3-galactosidase
In bifidobacteria, β-1,3-galactosidases are categorised as GH42 and GH35 and are responsible for cleaving HMOs possessing β-1,3 Gal linkages, displaying hydrolytic ability for both Type-I and Type-II chains with highest activity being displayed on LNT, followed by Lac, LNB and LNnT (James et al., Reference James, Motherway, Bottacini and van Sinderen2016; Yoshida et al., Reference Yoshida, Sakurama, Kiyohara, Nakajima, Kitaoka, Ashida, Hirose, Katayama, Yamamoto and Kumagai2012).
β-1,4-galactosidase
Several other glycosidases for the assimilation of HMOs exist, including β-1,4-galactosidases (Zeuner et al., Reference Zeuner, Teze, Muschiol and Meyer2019). In B. bifidum and B. breve, the β-1,4-galactosidases belong to the GH2 family and are categorised as BbgIII and LacZ2 and LacZ6, respectively, and are responsible for the hydrolysis of HMOs possessing Lac and Type-II chains (James et al., Reference James, Motherway, Bottacini and van Sinderen2016; Yoshida et al., Reference Yoshida, Sakurama, Kiyohara, Nakajima, Kitaoka, Ashida, Hirose, Katayama, Yamamoto and Kumagai2012). This mechanism of HMOs degradation is particularly prominent in B. bifidum and functions extracellularly cleaving LNnT at its Galβ-1,4 residue liberating Gal and LNT. Thereafter, LNT is further hydrolysed producing GlcNAc and lactose with lactose undergoing additional hydrolysis resulting in the formation of Glc and Gal, respectively (James et al., Reference James, Motherway, Bottacini and van Sinderen2016; Miwa et al., Reference Miwa, Horimoto, Kiyohara, Katayama, Kitaoka, Ashida and Yamamoto2010).
β-D-hexosaminidases
N-acetyl-β-D-hexosaminidases are another set of hydrolytic enzymes, which belong to the GH20 family with three enzymes seemingly responsible for the hydrolysis of HMOs. N-acetyl-β-D-hexosaminidases have been detected in B. longum subsp. infantis (Garrido et al., Reference Garrido, Ruiz-Moyano and Mills2012b) with these GHs being categorised as Blon_2355 and Blon_0732 and Blon_0459. Blon_2355 displays a preference for GlcNAc β1-3 Gal linkages, whereas Blon_0732 and Blon_0459 can additionally release GlcNAc from branched HMOs characterised by GlcNAc β1-6 Gal linkages (Garrido et al., Reference Garrido, Ruiz-Moyano and Mills2012b). Additionally, N-acetyl-β-D-hexosaminidases have been discovered in B. bifidum JCM 1254, categorised as BbhI and BbhII, with BbhI being shown to hydrolyse lacto-N-triose II into GlcNAc and lactose, respectively (Miwa et al., Reference Miwa, Horimoto, Kiyohara, Katayama, Kitaoka, Ashida and Yamamoto2010).
It is clear that different species, strains and subspecies of Bifidobacterium possess several different mechanisms for the assimilation of HMOs and it is likely that substantial differences in rates of consumption and fermentation occur.
The in vitro assimilation and consumption of HMOs
Differences in the consumption behaviour between different microorganisms found within in the gut microbiota have been assessed in vitro using either pooled or singular HMOs as sole carbon sources. To date, bifidobacteria are the most widely studied microorganisms, both singular and in combination, in relation to their ability to utilise HMOs due to their predominance in the breastfed infant gut microbiota. Yet, it is not only bifidobacteria that have the ability to degrade HMOs, several other genera within the gut, including Bacteroides, Roseburia and Akkermansia amongst others, appear to play a role in HMO utilisation.
Single cultures
As mentioned, bifidobacteria are one of the most well-documented microorganisms in the gut regarding HMO consumption. In one study, conducted by Gotoh et al. (Reference Gotoh, Katoh, Sakanaka, Ling, Yamada, Asakuma, Urashima, Tomabechi, Katayama-Ikegami, Kurihara, Yamamoto, Harata, He, Hirose, Kitaoka, Okuda and Katayama2018), the authors aimed to determine the ability of various strains of bifidobacteria to utilise HMOs, including 2’FL. The four strains of B. bifidum tested (JCM1254, JCM7004, TMC3108 and TMC3115) were isolated from either infant faecal samples or obtained from other researchers at the Riken Bioresource centre and subjected to in vitro assays using GAM broth and with sugar (HMOs) analysis and concentration being analysed in the spent media. There was a large amount of Fuc and Lac in the supernatants of all four B. bifidum strains assimilating both HMOs and Lac by 15 h, with the degradation of 2’FL beginning even before cells entered the exponential phase. This suggests that each strain of B. bifidum possessed the necessary 1,2-α-fucosidase and 1-3- and 1-4-α-fucosidases required for the degradation of fucosylated HMOs. Furthermore, the fermentation of 2’FL appeared to occur rapidly and extracellularly which may have important implications regarding the utilisation of HMOs within bifidobacterial communities and subsequent microbial diversity (Sakanaka et al., Reference Sakanaka, Gotoh, Yoshida, Odamaki, Koguchi, Xiao, Kitaoka and Katayama2020).
Garrido et al. (Reference Garrido, Ruiz-Moyano, Lemay, Sela, German and Mills2015) also examined the ability of several strains of B. longum subsp. infantis and B. bifidum utilised from breastfed infants to utilise HMOs, including 2’FL, 3’FL, 6’SL and LNT, as sole carbon sources. All B. longum subsp. infantis strains displayed excellent growth on all HMOs, while the ability of various B. bifidum strains was highly variable with several strains showing little growth on pooled HMOs. These findings are similar to those of Gotoh et al. (Reference Gotoh, Katoh, Sakanaka, Ling, Yamada, Asakuma, Urashima, Tomabechi, Katayama-Ikegami, Kurihara, Yamamoto, Harata, He, Hirose, Kitaoka, Okuda and Katayama2018), who recorded that the growth of B. bifidum strains (JCM1254, TMC3108 and TMC3115) resulted in higher cell biomass compared with B. bifidum strain JCM7004. There is clearly a remarkable variability in the ability of Bifidobacterium strains, even of the same species, to utilise HMOs with several species, strains and subspecies appearing to be better adapted than others (Sakanaka et al., Reference Sakanaka, Gotoh, Yoshida, Odamaki, Koguchi, Xiao, Kitaoka and Katayama2020).
The differences in the ability of Bifidobacterium to utilise HMOs were also demonstrated by Bunesova et al. (Reference Bunesova, Lacroix and Schwab2016), who tested several strains of Bifidobacterium, including B. longum subsp. infantis, B. longum subsp suis BSM11–5 and B. bifidum and Bifidobacterium kashiwanohense isolated from the stool samples of 6-month infants. There was substantial variability in the ability of bifidobacterial strains and subspecies to utilise HMOs with B. longum subsp. infantis being able to utilise 2′-FL, 3′-FL, 3′-SL and LNnT. B. bifidum BSM28-1 was able to utilise 6’SL in addition to 2′-FL, 3′-FL, 3′-SL and LNnT, while B. longum subsp. suis BSM11-5 and B. kashiwanohense strains all grew in the presence of 2′FL and 3’FL. Several strains and subspecies of bifidobacteria, however, including B. bifidum DSM20215 and B. breve DSM 20213, were unable to utilise HMOs to any real degree, with Bifidobacterium pseudolongum not being able to utilise HMOs at all. Furthermore, all B. longum strains and subspecies tested, including B. longum subsp. suis BSM11-5 and B. longum subsp. infantis DSM 20088, were able to utilise the resulting Fuc moiety, albeit with a varying degree of success with B. kashiwanohense not being able to metabolise Fuc at all. This adds to the evidence that the composition of the gut microbiota appears to be critical if HMOs are to be effectively utilised.
Ward et al. (Reference Ward, Ninonuevo, Mills, Lebrilla and German2007) also investigated the capability of Bifidobacterium to effectively degrade 2’FL and utilise the resulting Fuc and sialic acid moiety with B. longum bv. infantis ATCC 15697 achieving both the highest growth rate and the highest Fuc consumption amongst the majority of species and strains of Bifidobacterium tested, whereas B. breve ATCC 1570 was only able to achieve intermediate levels of growth with only moderate Fuc usage and B. adolescentis and B. bifidum ATCC 15696 exhibiting no growth on either Fuc or sialic acids. These findings are similar to those recorded by Garrido et al. (Reference Garrido, Ruiz-Moyano, Lemay, Sela, German and Mills2015), who noted that while B. longum subsp. infantis ATCC 15697 exhibited excellent growth on both 2’FL and 3’FL, B. bifidum JCM 7004 only exhibited moderate growth on 2’FL, 3’FL and 6’SL with B. animalis JCM 10602 exhibiting no growth, on HMOs at all. Accordingly, Locascio et al. (Reference LoCascio, Ninonuevo, Freeman, Sela, Grimm, Lebrilla, Mills and German2007) reported that B. adolescentis ATCC 15703 presented little-to-no sign of growth in the presence of several HMOs, whereas Xiao et al. (Reference Xiao, Takahashi, Nishimoto, Odamaki, Yaeshima, Iwatsuki and Kitaoka2010) found that B. adolescentis ATCC 15704 and 15705 appeared to be unable to utilise HMOs including LNB. This infers that B. adolescentis ATCC 15703, 15704 and 15705 lack the glycosidases and transporters required to assimilate HMOs (LoCascio et al., Reference LoCascio, Desai, Sela, Weimer and Mills2010).
The ability of the B. longum subsp. infantis ATCC 15697 to effectively utilise HMOs can be put down to the presence of five distinct gene clusters (Sela et al., Reference Sela, Chapman, Adeuya, Kim, Chen, Whitehead, Lapidus, Rokhsar, Lebrilla, German, Price, Richardson and Mills2008). In the genomic sequencing of B. longum subsp. infantis ATCC 15697 a 43-kb gene cluster dedicated to HMO import encoding 21 genes was identified with one of its four loci encompassing all of the necessary sialidases, fucosidases, galactosidases and hexosaminidases required for transporting and metabolising HMOs (LoCascio et al., Reference LoCascio, Ninonuevo, Freeman, Sela, Grimm, Lebrilla, Mills and German2007, Reference LoCascio, Ninonuevo, Kronewitter, Freeman, German, Lebrilla and Mills2009; Sela et al., Reference Sela, Chapman, Adeuya, Kim, Chen, Whitehead, Lapidus, Rokhsar, Lebrilla, German, Price, Richardson and Mills2008). Several other strains and subspecies of bifidobacteria, including B. longum subsp. longum DJO10AB and B. adolescentis ATCC 15703, possess fewer than 11 genes where the lack of SBPs results in an inability to effectively utilise HMOs (Lee et al., Reference Lee, Karamychev, Kozyavkin, Mills, Pavlov, Pavlova, Polouchine, Richardson, Shakhova, Slesarev, Weimer and O’Sullivan2008; LoCascio et al., Reference LoCascio, Ninonuevo, Kronewitter, Freeman, German, Lebrilla and Mills2009).
It is not just Bifidobacterium which possesses the ability to utilise and exhibit growth on HMOs. In addition to Bifidobacterium, several strains of Bacteroides, including B. fragilis and B. vulgatus, are also known prominent consumers of HMOs (Marcobal et al., Reference Marcobal, Barboza, Froehlich, Block, German, Lebrilla and Mills2010). Of these, two strains of B. fragilis ATCC2585 can effectively utilise a full range of HMOs, albeit displaying a preference for non-fucosylated HMOs, recording an overall consumption between 25 and 90 per cent. These findings are substantially higher than B. vulgatus ATCC8482, which can also utilise a full range of HMOs, again displaying a preference for fucosylated HMOs. This strain displayed much lower consumption rates of total HMOs than B. fragilis ATCC2585 at 16–40 per cent, respectively (Marcobal et al., Reference Marcobal, Barboza, Froehlich, Block, German, Lebrilla and Mills2010), the difference being the presence of Fuc-specific GH95 and GH29 glycoside hydrolases.
Furthermore, in contrast to Bifidobacterium and Bacteroides, several strains of Enterobacteriaceae, including EC1000, EC11775, EC29425 and SD13313, appear to be unable to utilise several HMOs, including 2‘FL and 6′SL while displaying limited growth on LNnT. However, these strains could also readily utilise Glc, maltodextrin and GOS as a sole carbon source in pure cultures (Hoeflinger et al., Reference Hoeflinger, Davis, Chow and Miller2015).
Mixed culture/faecal inoculum
The ability of 2’FL to alter the composition of the gut microbiota has been investigated using an in vitro Simulator of Human Intestinal Microbial Ecosystem (SHIME) model using faecal samples from 6-month-old infants had been exclusively formula-fed (Van den Abbeele et al., Reference Van den Abbeele, Duysburgh, Vazquez, Chow, Buck and Marzorati2019). The authors noted that 2’FL increased the relative abundance of bifidobacteria and butyrate-producing bacteria, shifting the distribution of Bifidobacterium spp. from B. bifidum towards B. adolescentis: an interesting finding given that, as previously discussed, B. adolescentis appears to be unable to utilise whole HMOs. This likely indicates that B. adolescentis can exploit products of the degradation of HMOs by other microbial community members (Thongaram et al., Reference Thongaram, Hoeflinger, Chow and Miller2017).
Increases in the concentration of acetate and butyrate were seen in both parts of the distal and proximal colon of the SHIME model, with levels of propionate displaying a more rapid increase in the distal part of the colon upon 2’FL dosing. This is consistent with Vester Boler et al. (Reference Vester Boler, Rossoni Serao, Faber, Bauer, Chow, Murphy and Fahey2013), who reported that 2’FL was rapidly fermented upon inoculation with mixed faecal cultures. However, the bifidogenic effect of 2’FL appeared to be donor-specific with increases in numbers of bifidobacteria only being observed in the proximal colon of one donor. In another, donor increases in bifidobacteria were observed in both the proximal and distal colon.
Additionally, the authors reported that increases in the concentration of acetate and butyrate detected were seen in both parts of the distal and proximal colon of the SHIME model with levels of propionate recording a more rapid increase in the distal part of the colon upon 2’FL dosing. Thus, from these results, it suggests that the microbiota dependence of individual rates of fermentation of 2’FL is likely to be highly variable between subjects (Marcobal and Sonnenburg, Reference Marcobal and Sonnenburg2012). Yet, the supplementation of 2’FL in this study was undertaken at 2 g/L, approximately twice the concentration of 2‘FL found in formula milks currently for sale on the market (SMA Nutrition, 2020), indicating that the results generated by this study may not give a fair reflection of what might transpire in real life.
In another study conducted by Salli et al. (Reference Salli, Anglenius, Hiryonen, Hibberd, Ahonen, Saarinen, Tiihonen, Maukonen and Ouwehand2019) using faecal samples from healthy infants aged below 1 year, the effects of 2’FL on the composition and metabolites of the infant microbiota were investigated using a semi-continuous colon simulator and was compared against GOSs with Lac as a control. Changes in microbial composition and metabolites were measured via 16S RNA amplicon sequencing and gas chromatography. From the results, it was noted that 2’FL recorded similar increases in numbers of total bacteria, Firmicutes and Actinobacteria (including bifidobacteria) compared to GOS, but 2’FL was unable to match GOS in reductions of numbers of Proteobacteria. Furthermore, levels of SCFAs and lactic acid produced by 2’FL were only half compared with those of GOS, suggesting that at least in this regard GOS results in a greater generation of metabolites associated with beneficial health outcomes than supplementation of 2’FL on its own.
Interestingly, Li et al. (Reference Li, Bauer, Chen, Wang, Kuhlenschmidt, Kuhlenschmidt, Fahey and Donovan2012) noted that in the in vitro fermentation of piglet faeces, LNnT recorded the largest increases in levels of acetate and butyrate compared with FOS, GOS/polydextrose mixture and pooled HMOs, whereas pooled HMOs and FOS recorded higher levels of propionate and lactate. Furthermore, both pooled HMOs and LNnT were able to stimulate changes in the microbial composition, including increasing numbers of total bacteria, Bifidobacterium, Lactobacillus, B. vulgatus and Clostridium cluster XIVa along with resulting in reductions in Clostridium cluster IV, suggesting that both pooled and single HMOs can drive beneficial changes in microbial composition. However, despite differences being detected in microbial composition, both pooled HMOs and LNnT appeared to be no more effective in stimulating changes in microbial composition compared with both FOS and the GOS/polydextrose mixture, respectively.
The metabolic by-products and fermentation characteristics of prebiotics, including GOS, 2’FL, LNnT, 6’SL, high-performance inulin (HP) and gum arabic, were investigated by Vester Boler et al. (Reference Vester Boler, Rossoni Serao, Faber, Bauer, Chow, Murphy and Fahey2013) using faecal samples isolated from both breast and formula-fed infants using an in vitro fermentation model. From the results, it was noted that the rates of fermentation of prebiotics differed significantly between breastfed and formula-fed infants inocula. For example, formula-fed inocula generated higher levels of acetate compared with breastfed inocula (P < 0.001) with 6’SL producing the largest concentration of acetate after 12-h fermentation. However, acetate production also varied over time between substrates with GOS generating large quantities of acetate regardless of diet. Butyrate appeared to be less affected by substrate or diet, but was higher in formula-fed inocula compared with breastfed inocula overall (P < 0.01); however, no differences were detected at any individual time point. Conversely, propionate was affected by diet, but more so by substrate and time with 6’SL producing large amounts of propionate after 12 h of fermentation. Moreover, the fermentation of 2’FL seemingly levelled off after 6 h, further indicating that 2’FL likely undergoes rapid fermentation upon inoculation (Salli et al., Reference Salli, Anglenius, Hiryonen, Hibberd, Ahonen, Saarinen, Tiihonen, Maukonen and Ouwehand2019). Finally, regarding microbial composition, numbers of bifidobacteria increased, whereas numbers of E. coli and Clostridium perfringens decreased regardless of the substrate used.
While using a pH-controlled in vitro fermentation model involving faecal donors from healthy, Irritable bowel syndrome (IBS) and ulcerative colitis patients, the most noticeable changes in gut microbiota composition were in Bifidobacterium (P < 0.01), while supplementation of 2’FL also resulted in increased numbers of Eubacterium rectale and Clostridium coccoides after 8- and 24-h fermentation [P < 0.01 (8 h) and P < 0.05 (24 h)] in healthy and [P < 0.01 (8 and 24 h)] IBD-ulcerative colitis donors. Significant increases in Roseburia at 8 h fermentation were seen in both healthy and IBS but not inflammatory bowel disease (IBD)-ulcerative colitis donors. Interestingly, in both IBD-ulcerative colitis and IBS patients but not healthy donors, there were significant increases in Atopobium cluster at 8 and 24 h (P < 0.01; Ryan et al., Reference Ryan, Monteagudo-Mera, Contractor and Gibson2021).
These results further add to the evidence that the ability of HMOs and 2’FL to stimulate changes in the microbiota and its resulting metabolites appears to be highly specific and restricted to certain species, strains and subspecies of microbes as well as the initial composition of the gut microbiota (Gotoh et al., Reference Gotoh, Katoh, Sakanaka, Ling, Yamada, Asakuma, Urashima, Tomabechi, Katayama-Ikegami, Kurihara, Yamamoto, Harata, He, Hirose, Kitaoka, Okuda and Katayama2018; Sakanaka et al., Reference Sakanaka, Gotoh, Yoshida, Odamaki, Koguchi, Xiao, Kitaoka and Katayama2020; Yu et al., Reference Yu, Chen and Newburg2013).
Cross-feeding: a strategy to ensure dominance?
As previously discussed, the gut microbiota, in particular bifidobacteria, have developed several strategies to colonise and dominate the microbiota of an infant’s gut with some strains, species and subspecies, displaying better potential than others. Interestingly, to help drive the colonisation of the gut, Bifidobacterium, Bacteroides as well as several other genera, including Akkermansia, Anaerostipes and Roseburia, have developed another strategy based on cross-feeding. Strains and species of Bifidobacterium and Bacteroides that are not able to utilise whole HMOs can feed on metabolites resulting from exploitation of HMOs by other species and strains (White et al., Reference White, Lamed, Bayer and Flint2014).
In breastfed infants, B. bifidum is said to make up of over 10 per cent of the total number of bifidobacteria present within their gut (Sakanaka et al., Reference Sakanaka, Hansen, Gotoh, Katoh, Yoshida, Odamaki, Yachi, Sugiyama, Kurihara, Hirose, Urashima, Xiao, Kitaoka, Fukiya, Yokota, Lo Leggio, Abou Hachem and Katayama2019). When B. bifidum is in abundance, the corresponding microbiota appears to follow suit with higher numbers of several other bifidobacterial species and strains also being recorded (Tannock et al., Reference Tannock, Lawley, Munro, Pathmanathan, Zhou, Makrides, Gibson, Sullivan, Prosser, Lowry and Hodgkinson2013). The potential for B. bifidum to act as cross-feeders for other members of the Bifidobacterium genus was noted by Asakuma et al. (Reference Asakuma, Hatakeyama, Urashima, Yoshida, Katayama, Yamamoto, Kumagai, Ashida, Hirose and Kitaoka2011), who documented that B. bifidum left several HMO components, including Fuc and Gal in spent media, indicating that extracellular degradation had occurred and suggesting that non-HMOs utilising species/subspecies may be able to exploit these monosaccharide moieties (Kitaoka, Reference Kitaoka2012).
Additionally, using faecal suspensions isolated from infants, children and adults in a mucin-based medium supplemented with HMOs, Egan et al. (Reference Egan, O’Connell Mtherway, Kilcoyne, Kane, Joshi, Ventura and van Sinderen2014) and Gotoh et al. (Reference Gotoh, Katoh, Sakanaka, Ling, Yamada, Asakuma, Urashima, Tomabechi, Katayama-Ikegami, Kurihara, Yamamoto, Harata, He, Hirose, Kitaoka, Okuda and Katayama2018) recorded the ability of several species and strains of bifidobacteria to grow in the presence and absence of B. bifidum. The overall findings of these studies suggest that in faecal suspensions possessing B. bifidum, the numbers of several bifidobacteria species and strains, including B. longum 105-A and B. breve UCC2003, increased; strains not known to effectively utilise whole HMOs to any real extent. It seems that B. bifidum is likely to be a prominent player in the establishment of the microbiota in early life (Kitaoka, Reference Kitaoka2012).
It was reported in a single ecosystem that 2′FL derived metabolites from B. pseudocatenulatum strains LH9, LH11, LH13 and LH14 supported the growth of several non-HMOs utilising strains including B. longum LH12. However, B. longum subsp. infantis LH23 2′FL degradation products did not support the growth of B. breve (LH21 and LH24), respectively. Additionally, with B. longum LH206 2′FL conditioned media, increases in numbers of all strains of B. longum subsp. infantis and B. pseudocatenulatum tested were seen. This indicates that the metabolism of 2′FL by B. infantis LH206 may generate a wider variety of growth-promoting compounds (Lawson et al., Reference Lawson, O’Neill, Kujawska, Javavdi, Wijeyesekera, Flegg, Chalklen and Hall2020).
It has been documented in a co-culture experiment that Anaerostipes cacae was able to utilise monosaccharides, as well as lactate and acetate, resulting from HMOs degradation by B. Infantis (Chia et al., Reference Chia, Mank, Blijenberg, Bongers, van Limpt, Wopereis, Tims, Stahl, Belzer and Knol2021). Roseburia spp. were able to grow in the presence of A. muciniphila, whereas in pure culture Roseburia spp. showed little-to-no sign of growth (Pichler et al., Reference Pichler, Yamada, Shuoker, Alvarez-Silva, Gotoh, Leth, Schoof, Katoh, Sakanaka, Katayama, Jin, Karlsson, Arumugam, Fushinobu and Abou Hachem2020). The ability of Bacteroides to act as primary degraders of HMOs was demonstrated in mice-fed sialylated HMOs, including 3’SL and 6’SL, when a marked increase in Enterobacteriaceae was seen. This led to an exacerbation of the pro-inflammatory response (Huang et al., Reference Huang, Chassard, Hausmann, von Itzstein and Hennet2015).
Additionally, in antibiotic-treated germ-free mice infected with either Salmonella typhimurium or C. difficile, it has been demonstrated that S. typhimurium was able to access both Fuc and sialic acid and C. difficile was able to readily utilise sialic acid as a result of breakdown of host carbohydrates by Bacteroides thetaiotaomicron (Ng et al., Reference Ng, Ferreyra, Higginbottom, Lynch, Kashyap, Gopinath, Naidu, Choudhury, Weimer, Monack and Sonnenburg2013). However, while the expansion of enteric bacterial pathogens via the utilisation of HMOs is sometimes seen in vitro in co-cultures or using in vivo mechanistic disease state rodent models, in the highly complex ecosystem in the human gut, this has never been reported.
Not all microorganisms found within the gut can participate in cross-feeding due to intracellular metabolism of polysaccharides/glycans. The inability of specific bifidobacteria to act as cross-feeders was demonstrated by Garrido et al. (Reference Garrido, Ruiz-Moyano, Kirmiz, Davis, Totten, Lemay, Ugalde, German, Lebrilla and Mills2016). B. longum SC596 exhibited excellent growth on both Type-I and Type-II chain HMOs, albeit displaying a preference for fucosylated HMOs; however, no monosaccharides from HMOs degradation were detected in the medium. As the degradation of HMOs by B. longum SC596 appears to be similar to that of B. longum subsp. infantis ATCC 15697, which uses intracellular metabolism (Garrido et al., Reference Garrido, Dallas and Mills2013), this suggests that B. longum SC596 cannot partake in the cross-feeding of other microorganisms.
These results suggest that the mutualistic behaviour which exists between microorganisms found in the gut likely influences the rates at which metabolites such as SCFAs are generated (Comstock, Reference Comstock2009). From this, one could conclude that the degree to which this mutualistic behaviour exists between microorganisms found in the gut not only increases the diversity of the gut microbiota, but is maybe one of the most critical characteristics in helping to shape a flexible, healthy ecosystem (Gotoh et al., Reference Gotoh, Katoh, Sakanaka, Ling, Yamada, Asakuma, Urashima, Tomabechi, Katayama-Ikegami, Kurihara, Yamamoto, Harata, He, Hirose, Kitaoka, Okuda and Katayama2018).
The influence of human milk oligosaccharides on infant microbiota composition in vivo
As previously discussed, the ability of HMOs to alter microbial composition in infants has been studied extensively using in vitro test conditions, but less so in vivo with only a limited number of studies being undertaken to date.
In a proof-of-concept study (De Leoz et al., 2015), serial faecal samples were collected from two vaginally born infants. Infant A was breastfed directly from birth, whereas infant B received formula supplementation for 4 days from days 2–6 and then was solely breastfed thereafter. Faecal samples were collected twice per week for the first month, twice per month in the second month and once or twice per month thereafter. Microbial compositions were analysed via 16S rRNA sequencing. The results demonstrated that after an initial rise in non-HMO-consuming bacteria, including Enterobacteriaceae and Staphylococcaeae, large shifts in microbial composition from non-HMO-consuming bacteria to HMO-consuming bacteria Bacteroidaceae and Bifidobacteriaceae were seen. Yet, large differences were seen between both donors whereby week 13 Bifidobacterium spp. dominated in infant A, and levels of most faecal HMOs dropped dramatically, whereas by week 14, Bacteroides spp. were most dominant in infant B.
Borewicz et al. (Reference Borewicz, Gu, Saccenti, Arts, Penders, Thijs, van Leeuwen, Lindner, Nauta, van Leusen, Schols and Smidt2019, Reference Borewicz, Gu, Saccenti, Hechler, Beijers, de Weerth, van Leeuwen, Schols and Smidt2020) aimed to correlate the HMOs in breast milk with changes in faecal microbiota composition, analysed via Illumina HiSeq 16S rRNA gene sequencing, in healthy 2-, 4-, 6- and 12-week-old breastfed infants. Unsurprisingly, the ability of infants to utilise HMOs, including 2’FL, was associated with differences in the faecal microbiota composition, with those infants possessing relatively high abundances of Bifidobacterium 418, 614 and 681 and Lactobacillus 744 (FDR < 0.05) reporting higher rates of 2’FL consumption. Additionally, infants who recorded higher consumptions rates of LNT and LNnT, LNFP III, LNFP II and lacto-N-hexaose (LNH) possessed significantly higher relative abundances of Bifidobacterium OTUs 418, 406, 643, 658, 423, 1335 and 597 and Bacteroides 144 (FDR < 0.05). Moreover, the degradation of sialylated HMOs 3′SL, 6′SL, LST a, LST b and LST c appeared to be more highly associated with Bacteroides; a finding confirming those reported by Yu et al. (Reference Yu, Chen and Newburg2013), who demonstrated using in vitro models that B. fragilis, B. vulgatus and B. thetaiotaomicron could utilise 3’SL and 6’SL as sole carbon sources.
Interestingly, Borewicz et al. ( Reference Borewicz, Gu, Saccenti, Arts, Penders, Thijs, van Leeuwen, Lindner, Nauta, van Leusen, Schols and Smidt2019, Reference Borewicz, Gu, Saccenti, Hechler, Beijers, de Weerth, van Leeuwen, Schols and Smidt2020) also recorded that lactobacilli appeared to thrive in the presence of 2′FL, DFL, LNDFH I, LNT, LNnT and LNFP II. This is fascinating given that it has been shown repeatedly in several in vitro studies that lactobacilli appear to be unable to utilise HMOs (Schwab and Ganzle, Reference Schwab and Ganzle2011; Ward et al., Reference Ward, Ninonuevo, Mills, Lebrilla and German2006). This suggests that lactobacilli might be able to thrive in the infant’s gut via cross-feeding, scavenging any resulting metabolites, including Fuc and lactose from the extracellular degradation of HMOs (Zuniga et al., Reference Zuniga, Monedero and Yebra2018). This likely infers that the degradation of HMOs strongly correlates with the microbiota and specifically with the relative abundance of the phylotypes Bifidobacterium, Bacteroides and Lactobacillus present within an infant’s gut.
Moreover, in a randomised, double-blind, multicentre clinical trial, healthy full-term infants (aged 0–14 days) were fed infant formula with no added HMOs (control), or the same formula with the addition of 2’FL and LNnT for a timeframe of 6 months. Thereafter, all infants were fed the same non-HMO-containing infant formula (Berger et al., Reference Berger, Porta, Foata, Grathwohl, Delley, Moine, Charpagne, Siegwald, Descombes, Alliet, Puccio, Steenhout, Mercenier and Sprenger2020). Results were analysed against a breastfed reference group with changes in microbial community types being analysed at 3 and 12 months via 16S rRNA gene sequencing. The results indicated that, compared with the breastfed reference group, the HMO-containing formula stimulated increases in Bifidobacterium, albeit to a lower degree than the reference breastfed group. Levels of Escherichia were, however, significantly lower in the HMO-containing formula group compared with the control group and were similar to those in the breastfed group. Numbers of Peptostreptococcaceae were far higher in the control and HMO-containing formula group compared with the breastfed group. Yet, at 12 months, no differences were detected between the two formula groups. This suggests that the supplementation of infants with HMO-containing formulae may offset some of the ill effects associated with not breastfeeding from birth. However, this study is not without limitation. First, only two faecal samples were collected: one at 3 months and one at 12 months. Second, no data were collected on day-care attendance and when solid foods were introduced (weaning) which, due to the effects these factors have on the microbial composition (McBurney et al., Reference McBurney, Davis, Fraser, Schneeman, Huttenhower, Verbeke, Walter and Latulippe2019), may have introduced biases into the results. Consequently, further investigation into this area would be highly beneficial to determine the true effects that both 2’FL and LNnT have on altering the composition of healthy infants in vivo.
In another study, differences in gut microbiota composition between caesarean and vaginally born babies of α1-2 fucosylated secreting mothers were conducted by Tonon et al. (Reference Tonon, Morais, Taddei, Araujo, Abrao, Miranda and de Morais2021). In this study, faecal microbiota composition from caesarean and vaginally born infants was analysed by 16S rRNA gene sequencing and qPCR with results being stratified by secretor status. The authors concluded that levels of Bifidobacterium were similar between caesarean and vaginally born infants of secretor mothers. Yet, there were differences between caesarean and vaginally born infant microbiotas with the caesarean born infants from secretors possessing higher amounts of Kluyvera and Veillonella. Vaginally born infants from secretor mothers possessed higher amounts of Bacteroides. This further adds to the evidence that mode of delivery may likely impact on proliferation of the gut microbiota and HMO utilisation.
In addition to healthy infant’s, the effects of HMOs on the gut microbiota and health outcomes also been studied in preterm infants. In one study, 12 premature infants were randomised into two groups – one group containing formula and increasing doses of short-chain GOSs (degree of polymerisation < 8) and the other group receiving formula + HMOs (Underwood et al., Reference Underwood, Kalanetra, Bokulich, Mirmiran, Barile, Tancredi, German, Lebrilla and Mills2014). The authors noted that relative abundances of clostridia increased with increasing doses of HMOs. The authors also noted that there were trends towards increase of γ-proteobacteria over time/dose in preterm infants feed formula + HMOs.
Additionally, in a study involving preterm infants with necrotising enterocolitis (NEC), it was noted that infants with NEC possessed higher levels of Proteobacteria and lower levels of Actinobacteria at phylum levels, along with lower relative abundances of B. longum and higher relative abundances of Enterobacter cloacae. The authors also noted that the composition of breast milk, specifically lower concentration of DSLNT, was associated with the likelihood of developing NEC (Masi et al., Reference Masi, Embleton, Lamb, Young, Granger, Najera, Smith, Hoffman, Petrosino, Bode, Berrington and Stewart2021). While, similarly, Underwood et al. (Reference Underwood, Gaerlan, De Leoz, Dimapasoc, Kalanetra, Lemay, German, Mills and Lebrilla2015) concluded that preterm infants of non-secretor mothers possessed higher levels of Proteobacteria and lower levels of Firmicutes, secretor mothers possess specific fucosylated HMOs including LDFT and LNFP V, which may be associated with lower levels of Enterobacteriaceae and potentially protective effect against pathogens associated with NEC. These results potentially infer that the composition of HMOs present in breast milk may be a contributing factor towards the development of NEC in preterm infants.
Moreover, in another study conducted in healthy rats, Chleilat et al. (Reference Chleilat, Klancic, Ma, Schick, Nettleton and Reimer2020) investigated the effects that the supplementation of 2′FL and 3′SL either together or on their own had on microbial composition compared to a non-HMOs control. In general, all HMO-fortified diets altered gut microbiota composition. However, larger increases in Bifidobacterium spp. were recorded in the 2′FL group compared with the 3′SL-fortified group (P = 0.03). Additionally, A. muciniphila numbers were significantly lower in 3′SL + 2′FL group compared with the control (P < 0.01), respectively.
In a study involving mice supplemented with the 2’FL and 2’FL consuming strain B. pseudocatenulatum MP80, it was noted that 2’FL created an environment that allowed B. pseudocatenulatum MP80 to thrive, along with finding that 2’FL increased the Bifidobacteriaceae relative abundance, as well as resulting in higher log ratios of Bacteroidaceae and Bifidobacteriaceae relative to Lachnospiraceae and Ruminococcaceae amplicon sequencing variant (P = 0.003).
These results help to explain the large variability in the presence and levels of HMOs detected in the faecal samples of infants, even when secretor status is considered, with virtually no HMOs being detected in faecal samples of several infants, whereas, in the faecal samples of other infants, there was a strong presence of non-fucosylated HMOs, suggesting the presence of the fucoside-utilising microorganisms needed to degrade fucosylated HMOs (Asakuma et al., Reference Asakuma, Hatakeyama, Urashima, Yoshida, Katayama, Yamamoto, Kumagai, Ashida, Hirose and Kitaoka2011). In faecal samples of several other infants, large quantities of LNnT were detected with LNnT not being detected in others. However, despite these differences, a common characteristic amongst nearly all infants used in these studies was what appeared to be the presence of several new, non or partially intact HMOs and HMOs by-products in faecal samples with the majority of new HMOs detected displaying a high proportion of HexNAc (Davis et al., Reference Davis, Totten, Huang, Nagshbandi, Kirmiz, Garrido, Lewis, Wu, Smilowitz, German, Mills and Lebrilla2016; De Leoz et al., Reference De Leoz, Wu, Strum, Ninonuevo, Gaerlan, Mirmiran, German, Mills, Lebrilla and Underwood2013; Dotz et al., Reference Dotz, Rudloff, Meyer, Lochnit and Kunz2015).
Thus, while results seemingly imply that the supplementation of 2’FL and LNnT may contribute towards a positive shift in the composition of the gut microbiota, in reality, the make-up of the infant’s microbiota is shaped through several often complex and interacting factors from birth, including mode of delivery (vaginal vs. c-section), feeding practices (breast vs. bottle feeding) and age at which the introduction of complex dietary substrates occurs (weaning). The use of gut microbiome altering medications and supplements, namely antibiotics and probiotics (Bertelsen et al., Reference Bertelsen, Jensen and Ringel-Kulka2016; McBurney et al., Reference McBurney, Davis, Fraser, Schneeman, Huttenhower, Verbeke, Walter and Latulippe2019), will also have an impact. Consequently, the degradation of HMOs will differ greatly depending on the relative abundance of specific species, strains and subspecies of microorganisms present within an individual infant’s gut microbiome. More detailed analysis is needed for infant microbiota prior to supplementation in such studies. The supplementation of 2’FL and LNnT in infant formula milk may be of little-to-no benefit to many infants as several infants especially those who were never breastfed may not possess the necessary microorganisms and thus glycosidases and transporters needed to effectively utilise these specific HMOs.
Acknowledgement
Peter Jackson would like to thank Prof Bob Rastall and Dr Anisha Wijeyesekera for their support and feedback in writing this manuscript.
Disclosure statement
No potential conflicts of interest were reported by the authors.
Research transparency and reproducibility
The opinions/views contained within this article are those of the authors.
Author contributions
Conceptualisation: P.P.J.J, R.A.R and A.W; Formal analysis: P.P.J.J.; Funding acquisition: R.A.R.; Investigation: P.P.J.J.; Methodology: all authors; Project administration: R.A.R.; Supervision: R.A.R and A.W.; Visualisation: all authors; Writing – original draft: P.P.J.J.; Writing – review and editing: all authors. P.P.J.J. drafted and revised all manuscript drafts following feedback from co-authors R.A.R and A.W.
Funding
This research received no specific grant from any funding agency, commercial or not-for-profit sectors.