Introduction
Information on organisms from extreme terrestrial environments could contribute to the question on the origin of life on Earth. It might be possible that life arose and persisted in such environments (Rothschild and Mancinelli, Reference Rothschild and Mancinelli2001), and then dispersed to different corners of the universe (Arrhenius, Reference Arrhenius1908; Cavicchioli, Reference Cavicchioli2002). Microorganisms were shown to survive impacts of low velocity (about 1–2 km s−1) in exposure experiments (Horneck et al., Reference Horneck, Stöffler, Ott, Hornemann, Cockell, Moeller, Meyer, De Vera, Fritz, Schade and Artemieva2008), which confirms the possibility of transmission of simple unicellular organisms from one planet to another.
Mars is considered to be one of the most favourable places in the Solar System to search for past and present life. Currently, Mars is inhospitable for terrestrial microbes due to low temperatures, high concentrations of salts, especially perchlorates, UV radiation and low content of organic compounds (Berry et al., Reference Berry, Jenkins and Schuerger2010). However, we may expect that organics were much more abundant on early Mars, when lakes or even oceans were present on Martian surface as suggested by several scientists (Head et al., Reference Head, Hiesinger, Ivanov, Kreslavsky, Pratt and Thomson1999; Carr and Head, Reference Carr and Head2003; Dohm et al., Reference Dohm, Baker, Boynton, Fairén, Ferris, Finch, Furfaro, Hare, Janes, Kargel, Karunatillake, Keller, Kerry, Kim, Komatsu, Mahaney, Schulze-Makuch, Marinangeli, Ori, Ruiz and Wheelock2009).
It is conceivable that Mars could be contaminated with life by meteoroids of terrestrial origin (Fairén and Schulze-Makuch, Reference Fairén and Schulze-Makuch2013). Halophiles can be considered promising for studying the potential to live on Mars, since only salt solutions can exist there as liquids. In the past Mars was warmer and wetter (e.g. Clifford and Parker, Reference Clifford and Parker2001), so terrestrial halophiles can be regarded as analogues of hypothetical ancient Martian halophiles. Recent studies demonstrated the presence of magnesium perchlorate, magnesium chlorate, sodium perchlorate and contemporary water activity on Martian surface (Ojha et al., Reference Ojha, Wilhelm, Murchie, McEwen, Wray, Hanley, Massé and Chojnacki2015), so interest in terrestrial halophiles is again increasing. Halophiles are found in multiple environments on Earth, including saline rocks dated back to 200–250 Mya (Mancinelli et al., Reference Mancinelli, Fahlen, Landheim and Klovstad2004), saline surface waters, such as the Dead Sea, Lake Magadi and other salt lakes in various arid zones of our planet (Oren, Reference Oren2002). Astrobiological importance of halophiles and hypersaline environments was already investigated in several studies (DasSarma, Reference DasSarma2006; Gunde-Cimerman et al., Reference Gunde-Cimerman, Oren and Plemenitaš2006; Mormile et al., Reference Mormile, Hong and Benison2009). Many papers focused on the chances of terrestrial halophiles to survive on Mars and other extraterrestrial environments (Litchfield, Reference Litchfield1998; Landis, Reference Landis2001; Cavicchioli, Reference Cavicchioli2002; Mancinelli et al., Reference Mancinelli, Fahlen, Landheim and Klovstad2004; Reid et al., Reference Reid, Sparks, Lubow, McGrath, Livio, Valenti, Sowers, Shukla, MacAuley, Miller, Suvanasuthi, Belas, Colman, Robb, DasSarma, Müller, Coker, Cavicchioli, Chen and DasSarma2006; Oren et al., Reference Oren, Bardavid and Mana2014).
Biochemical adaptation of halophilic archaea to high-salt environment, which could be present on early Mars, was considered by Litchfield (Reference Litchfield1998). The impact of microgravity simulating interplanetary flights on survival of halophilic archaea was studied by Dornmayr-Pfaffenhuemer et al. (Reference Dornmayr-Pfaffenhuemer, Legat, Schwimbersky, Fendrihan and Stan-Lotter2011). Halophilic microorganisms were able to survive under the CO2-dominated Martian atmosphere at high temperatures (Leuko et al., Reference Leuko, Weidler, Radax, Legat, Komle, Kargl and Stan-Lotter2002). Some terrestrial halophiles were able to grow at low temperatures up to −1 °C (Reid et al., Reference Reid, Sparks, Lubow, McGrath, Livio, Valenti, Sowers, Shukla, MacAuley, Miller, Suvanasuthi, Belas, Colman, Robb, DasSarma, Müller, Coker, Cavicchioli, Chen and DasSarma2006) and survive launches into the stratosphere, with conditions similar to the surface of Mars (DasSarma et al., Reference DasSarma, Laye, Harvey, Reid, Shultz, Yarborough, Lamb, Koske-Phillips, Herbst, Molina, Grah, Phillips and DasSarma2017).
Each new study adds to the information on the potential of microorganisms to survive under extraterrestrial conditions and on the origin of life. Therefore, biodiversity of extremophiles, including halophiles, could have implications for the search for Martian life. In this study we investigate microbial communities of the poorly studied Kulunda steppe located in the Altai region (Russia). The South of West Siberia (which includes the Altai Krai) contains over 20 000 lakes of different sizes and salinity (Strakhovenko et al., Reference Strakhovenko, Roslyakov, Syso, Ermolaeva, Zarubina, Taran and Puzanov2016); lakes make up 8.6% of this area, compared with the average of 4.0% for Russia (Ermolaev and Vizer, Reference Ermolaev and Vizer2010). This region is highly continental, with low and unstable precipitation. Numerous lakes in the region are likely to represent the remains of an ancient sea, as they are localized within the paleosea or larger paleolakes (Kuz'mina et al., Reference Kuz'mina, Volkova, Gnibidenko and Lebedeva2003; Akhmet'ev, Reference Akhmet'ev2011; Iakovleva, Reference Iakovleva2011). These drainless lakes have high salt content (up to the saturation point), and pH as high as 10 (Ermolaev and Vizer, Reference Ermolaev and Vizer2010), and the majority of them are shallow (average depth 2–3 m) and small (area up to 2.5 km2) (Beirom et al., Reference Beirom, Vasiliev and Gadzhiev1986). Small lakes receive sufficient sunlight and biogenic elements, so they are one of the most productive mineralized water ecosystems. They are characterized by a deficit of oxygen in winter and accumulate sapropel sediments (Strakhovenko et al., Reference Strakhovenko, Roslyakov, Syso, Ermolaeva, Zarubina, Taran and Puzanov2016). Studies performed for several lakes of the studied region suggest that their microbiology and geochemistry are unique (Vesnina et al., Reference Vesnina, Mitrofanova and Lisitsyna2005; Kompantseva et al., Reference Kompantseva, Komova, Rusanov, Pimenov and Sorokin2009; Sorokin et al., Reference Sorokin, Zacharova, Pimenov, Tourova, Panteleeva and Muyzer2012; Bryanskaya et al., Reference Bryanskaya, Malup, Lazareva, Taran, Rozanov, Efimov and Peltek2016). Altai saline lakes are used as sources of chemical compounds, food (various organisms), as well as for recreation, with silts of certain lakes particularly useful for balneology (Dolmatova, Reference Dolmatova2010).
The main goal of this study was to investigate the potential of bacteria and archaea from certain Altai salt lakes to survive low temperatures, high salt and metal concentrations, as well as the possibility of living on various organic substrates.
Materials and methods
Sampling locations and strain isolation
Samples were taken from upper layers of bottom sediments in the littoral zones of the following lakes in the Altai Krai: Burlinskoe, Bolshoe Yarovoe, Maloe Yarovoe, Kulundinskoye, Gorkoye (Romanov district), Solenoye, Krivoye, Belenkoye, etc. Microbes were cultivated at 37 °C in the base growth medium containing 5 g l−1 MgCl2·6H2O, 1 g l−1 KCl, 1 g l−1 CaCl2·2H2O, 200–250 g l−1 NaCl, 4 g l−1 tryptone, 2 g l−1 yeast extract and 10 ml l−1 microelement solution (mg l−1: 700 FeSO4·7H2O, 234 CoCl2·6H2O, 24 Na2MoO4·2H2O, 33 NaWO4·2H2O, 100 ZnSO4·7H2O, 5 CuSO4, 10 H3BO3, 120 MnSO4·5H2O); pH was adjusted to 7.5 in all cases. Microbial strains were isolated only from samples from five lakes (Burlinskoe, Bol'shoe Yarovoe, Maloe Yarovoe, Kulundinskoye and Gorkoye), with mineralization ranging from 94 to 346 g l−1 (Zarubina and Durnikin, Reference Zarubina and Durnikin2005; Lebedeva et al., Reference Lebedeva (Verba), Lopukhina and Kalinina2008) (see Table 1). The salinity of the base growth medium corresponds to salinity of studied Altai lakes (see Table 4).
Table 1. Sampling locations and some environmental variables of the studied Altai lakes (Leonova et al., Reference Leonova, Bogush, Bobrov, Bychinskiy, Trofimova and Malikov2007; Lebedeva et al., Reference Lebedeva (Verba), Lopukhina and Kalinina2008; Strakhovenko et al., Reference Strakhovenko, Malikova and Ustinov2013)
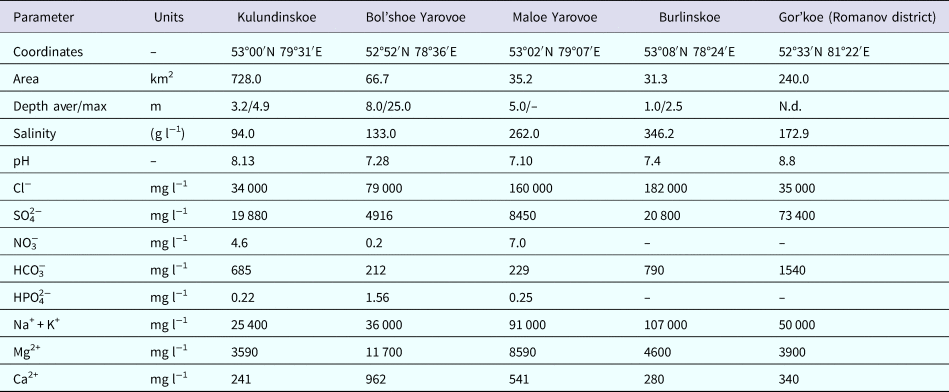
We isolated a total 20 strains for collection of biotechnological microorganisms as a source of novel promising objects for biotechnology and bioengineering of Federal Research Center ‘Institute of Cytology and Genetics of the Siberian Branch of the RAS’, 11 of which demonstrated stable growth and were included in a subsequent work.
16S rRNA gene amplification and sequencing
DNA was extracted using a Genome DNA Purification kit (Fermentas). Universal primers Arch22F (5′-ATTCCGGTTGATCCTGC) and UA1406R (5′-ACGGGCGGTGWGTRCAA) were used. For sequencing, primers ARh915r (5′-GTGCTCCCCCGCCAATTCCT), Arch22F (5′-ATTCCGGTTGATCCTGC) and UA1406R (5′-ACGGGCGGTGWGTRCAA) were used. Capillary electrophoresis of sequencing reactions was performed in the DNA Sequencing Center of Collective Usage ICG SB RAS (Novosibirsk). Sequencing results were visualized using Sequence Scanner 1.0.
BLAST algorithms from the NCBI site were used to search for closely related sequences in the refseq_RNA database. Phylogenetic trees were constructed using the neighbour-joining algorithm in the MEGA 6.06 program. Branch support was calculated using the bootstrap method.
The GenBank/EMBL/DDBJ accession numbers for the 16S rRNA gene sequences of strains are KX911478–KX911489.
Cultivation of microorganisms
Exposure experiments were performed on the base growth medium for 7–21 days. At least three exposure experiments were performed for each strain. Cell number was estimated based on cfu number.
Freeze–thaw cycles
In order to estimate survival potential of the studied strains we performed the following experiments: (1) single freezing and thawing at various temperatures and NaCl concentrations and (2) two freeze–thaw cycles under the same conditions.
Cultures were placed in the liquid base growth medium at −18 and −70 °C for 7 days for each freeze–thaw cycle. Cryoconservation was performed in 2 ml Eppendorf tubes at −70 °C in an Ultra-Low Temperature Freezer, New Brunswick Scientific, USA. Thawing was performed by air convection at +25 °C. The temperature changing rate during defrosting was about 9° min−1 for temperatures between −70 and −21 °C and about 2° min−1 at temperatures between −21 and 0 °C. Then the culture was defrosted, transferred to solid base growth medium and incubated at 37 °C. The survival rate of strains in each freeze–thaw cycle was calculated as the ratio of cfu of exposed cells to that of control cells.
Physicochemical properties of salt solutions including pH, ice fraction and composition of solid-state species was performed using the FREZCHEM program (Marion et al., Reference Marion, Catling and Kargel2003).
Microscopy
The morphology of cells and the state of cell walls before and after freezing were determined using light and electron microscopy in the Center of Collective Usage for Microscopic Analysis of Biological Objects SB RAS. Samples were prepared using conventional protocols (Netrusov et al., Reference Netrusov, Egorova, Zakharchuk, Kolotilova, Kotova, Semenova, Tatarinova, Ugol'nikova and Tsavkelova2005).
Growth on media with various chemical compositions
We studied three strains, one bacterial (Halomonas sp. Н12b) and two archaean ones (Halorubrum saccharovorum Н3a and Halorubrum sp. Н7a), that demonstrated a high survival rate after two freeze–thaw cycles and various NaCl concentrations, and at the same time were sufficiently sensitive to environmental conditions (Bryanskaya et al., Reference Bryanskaya, Berezhnoy, Rozanov, Peltek and Pavlov2013).
The base growth medium was used as the control. The content of experimental media is given in Table 2.
Table 2. The composition of experimental media

Growth on various organic substrates
Urease and β-galactosidase activity, citrate utilization, esculin hydrolysis, acid production and growth on various carbohydrates were assessed according to Netrusov et al. (Reference Netrusov, Egorova, Zakharchuk, Kolotilova, Kotova, Semenova, Tatarinova, Ugol'nikova and Tsavkelova2005) and Logan and De Vos (Reference Logan, De Vos, De Vos, Garrity, Jones, Krieg, Ludwig, Rainey, Schleifer and Whitman2009). Most of the tests were performed using reagents and kits produced by Lachema, DIA-M and Sigma. All tests were performed in triplicate.
Results
Phylogenetic affinities of the studied strains
We performed 16S rRNA sequencing for 12 strains: nos. 1–9 and 11–13. Five of the obtained sequences were identified as bacteria belonging to the genera Pseudomonas (H5b), Halomonas (Н6b, H12b), Salicola (H8b, H9b) and seven, to Archaea from the genera Haloarcula (H1a) and Halorubrum (H2a, H3a, H4a, H7a, H11a, H13a) (Fig. 1, Table 3).

Fig. 1. Phylogenetic tree based on 16S rRNA sequences constructed using the neighbour-joining algorithm. Numbers indicate bootstrap support values. Bar, 0.1 changes per nucleotide position.
Table 3. Sampling locations and taxonomic positions of the studied microorganisms

All 12 strains belonged to halophiles (Oren, Reference Oren2002); seven, to extremely halophilic archaea, and four, to bacteria. Six of the seven archaeal strains belonged to the genus Halorubrum, widespread and abundant in many halophilic ecosystems (Corral et al., Reference Corral, De la Haba, Sánchez-Porro, Amoozegar, Papke and Ventosa2015). Complete genome of Halorubrum sp. H3a strain was already sequenced by our group (Rozanov et al., Reference Rozanov, Bryanskaya, Malup, Kotenko and Peltek2015), which allowed us to assign this strain to H. saccharovorum. The genome of cold-adapted haloarchaeon Halorubrum lacusprofundi has also been completely sequenced and its derived protein sequences analysed bioinformatically (DasSarma et al., Reference DasSarma, Capes, Karan and DasSarma2013; Anderson et al., Reference Anderson, DasSarma, Lucas, Copeland, Lapidus, Del Rio, Tice, Dalin, Bruce, Goodwin, Pitluck, Sims, Brettin, Detter, Han, Larimer, Hauser, Land, Ivanova, Richardson, Cavicchioli, DasSarma, Woese and Kyrpides2016). The remaining archaean strain belonged to the less studied genus Haloarcula with nine validly described species (Minegishi et al., Reference Minegishi, Kamekura, Kitajima-Ihara, Nakasone, Echigo, Shimane, Usami, Itoh and Ihara2012).
Two of the bacterial strains belonged to the extremely halophilic genus Salicola, first described in 2006 (Proteobacteria type, Gammaproteobacteria class), with only two valid species (Kharroub et al., Reference Kharroub, Aguilera, Quesada, Morillo, Ramos-Cormenzana, Boulharouf and Monteoliva-Sánchez2006; Maturrano et al., Reference Maturrano, Valens-Vadell, Rosselló-Mora and Antón2006). The rest were identified as representatives of Halomonas, a widespread and abundant genus with over 90 species (Parte, Reference Parte2014).
Haloarcula sp. H1a, Pseudomonas sp. H5b and Halomonas sp. H6b demonstrated unstable growth and were thus excluded from further experiments.
The impact of NaCl, temperature and freeze–thaw cycles on bacterial survival
The studied bacteria could grow at a wide range of NaCl concentration (50–300 g l−1). However, growth parameters varied for different strains. Salicola sp. Н8b demonstrated a decreased growth rate at 50 and 300 g l−1 NaCl, and the lowest salt concentration had more pronounced effect than the highest one (Fig. 2(a) and (b)). At 100 and 200 g l−1 NaCl, initial number of cfu was high at all studied temperatures and decreased with increasing cycle number. Salicola sp. Н9b had low cfu at 50 g l−1 NaCl and failed to survive freezing (Fig. 2(c) and (d)). At 100 and 200 g l−1 NaCl, cfu values were high in all experiments, but decreased significantly after a freeze–thaw cycle. At 300 g l−1 NaCl, initial cfu values were as high as at 100 and 200 g l−1, but cells failed to survive initial freezing at −70 °C and second freezing at −18 °C.

Fig. 2. Bacterial growth after exposure to various NaCl concentrations and freeze–thaw cycles: Salicola sp. H8b, −70 °C (a) and −18 °C (b); Salicola sp. H9b, −70 °C (c) and −18 °C (d); Halomonas sp. H12b, −70 °C (e) and −18 °C (f).
Halomonas sp. H12b demonstrated high initial cfu values in all experiments except for 300 g l−1 NaCl, when initial cfu values were low and cells failed to survive freezing (Fig. 2(e) and (f)). A second freeze–thaw cycle reduced cfu number for this strain, most notably at −70 °C and 50 g l−1 NaCl.
For archaeal strains, the same NaCl concentrations were tested; all strains failed to grow below 200 g l−1 NaCl. No archaeal strain could survive freezing at −70 °C and 300 g l−1 NaCl. The Halorubrum sp. H7 strain survived a single freezing at −18 °C and 300 g l−1 NaCl, while H. saccharovorum H3a withstood two cycles under such conditions (Fig. 3). Freeze–thaw cycles dramatically reduced cfu number in most experiments. Let us note that survival rates at 200 g l−1 NaCl after freezing at −18 and −70 °C were comparable for all strains except for H11a (see Fig. 3).

Fig. 3. Growth of archaeal strains exposed to freeze–thawing and various NaCl concentrations: Halorubrum sp. H2а, −70 °C (a) and −18 °C (b); Halorubrum saccharovorum H3а, −70 °C (c) and −18 °C (d); Halorubrum sp. H4а, −70 °C (e) and −18 °C (f); Halorubrum sp. H7а, −70 °C (g) and −18 °C (h); Halorubrum sp. H11а, −70 °C (i) and −18 °C (j); Halorubrum sp. H13а, −70 °C (k) and −18 °C (l).
The impact of freezing temperature on the survival rate
Freezing impacts cells via low temperatures, crystallization of solid-state fraction and by stresses arising due to changes in ice density. To study these effects separately, several experiments at different temperatures of partial freezing were performed. All studied media remained partly frozen at −18 °C except for 300 g l−1 NaCl solution (Fig. 4), which was totally liquid. Temperature varied within 1 °C, which corresponds to small changes in ice fraction (Fig. 4).

Fig. 4. Fraction of liquid phase in the NaCl - water system versus temperature and NaCl content (the content of minor compounds: 1 - KCl, 1 - CaCl2, 5 - MgCl2 (in g l−1)). The numbers 50, 100, 200 and 300 denote the NaCl content in the studied media (in g l−1).
The ratio of survival fractions at −70 and −18 °C rapidly increases with increasing of survival fraction at −70 °C (Fig. 5). Survival fractions of strains Halorubrum sp. H4a and Halorubrum sp. H7a are the most sensitive to changes of temperature from −18 to −70 °C. The survival rate in partially frozen media decreased, and increase in cfu number was observed after freezing. Survival of these strains is higher for completely frozen solution in comparison with partly frozen solution.

Fig. 5. Survival rates of Halorubrum sp. H4a and Halorubrum sp. H7a at low temperatures in freeze–thawing experiments.
Electron microscope images show that low temperatures led to destruction of cell membranes of strains demonstrating only partial survival after freeze–thaw cycles, while membranes of those resistant to −70 °C were damaged (Fig. 6). Thus, a decrease in survival rate is probably caused by destruction of cell membranes (Fig. 6).

Fig. 6. Electron microscopic images of (a) Halomonas sp. H12b before freezing; (b) Halomonas sp. H12b, (c) Halorubrum sp. H4a and (d) Halorubrum sp. H7a after freezing at −70°С.
Dependence on anion and cation content
Geochemical composition of five studied lakes of the Altai Krai (Burlinskoye, Gorkoye, Bolshoe Yarovoe and Maloe Yarovoe) was determined by Leonova et al. (Reference Leonova, Bogush, Bobrov, Bychinskiy, Trofimova and Malikov2007); Lebedeva et al. (Reference Lebedeva (Verba), Lopukhina and Kalinina2008); Strakhovenko et al. (Reference Strakhovenko, Malikova and Ustinov2013) and for 11 lakes of the Novosibirsk oblast, by Bryanskaya et al. (Reference Bryanskaya, Malup, Lazareva, Taran, Rozanov, Efimov and Peltek2016) (Table 4).
Table 4. Chemical composition of saline lakes of the Altai and Novosibirsk regions and media used in this study (Leonova et al., Reference Leonova, Bogush, Bobrov, Bychinskiy, Trofimova and Malikov2007; Lebedeva et al., Reference Lebedeva (Verba), Lopukhina and Kalinina2008; Strakhovenko et al., Reference Strakhovenko, Malikova and Ustinov2013; Bryanskaya et al., Reference Bryanskaya, Malup, Lazareva, Taran, Rozanov, Efimov and Peltek2016).

a Not measured, estimated value assuming the same enrichment as Cl− enrichment in comparison with the standard mean ocean water at 3.5 wt % salinity (Turekian, Reference Turekian1968).
Water of the studied lakes belonged to chloride and chloride-sulphate types (Zarubina and Durnikin, Reference Zarubina and Durnikin2005; Lebedeva et al., Reference Lebedeva (Verba), Lopukhina and Kalinina2008). Based on the comparison of the base growth medium and water composition of the studied lakes we decreased metal cation content (Fe2+, Co2+, Mn2+), added the previously absent anions (CO32−, NO3−, HPO42−) and increased BO33− content (from 0.1 to 3.1 mg l−1).
In experiment 1, we studied growth of three strains (H. saccharovorum H3a, Halorubrum sp. H7a, Halomonas sp. H12b) on media with different concentrations of anions and metals. The results indicate that Halomonas sp. H12b is the most tolerant to variations in medium composition (Fig. 7), and both increase in anion concentration and decrease in metal content led to a slight increase in cfu number.

Fig. 7. Results of experiment 1: growth of three strains (H. saccharovorum H3a, Halorubrum sp. H7a, Halomonas sp. H12b) in media with different concentrations of anions and cations.
Figure 7 demonstrates that cfu number of Halomonas sp. H12b did not depend on the presence or absence of the studied anion concentrations (carbonates, nitrates, phosphates, borates) in medium, while lower content of microelements was more favourable.
For the archaean H. saccharovorum Н3а, the number of cfu increased with decreased content of metal ions, while addition of anions and decrease of metal ion content led to a decreased cfu number. Those effects were even more pronounced in Halorubrum sp. Н7а: at decreased content of metal ions cfu number was twice as high relative to control, while addition of anions resulted in fourfold decrease in cfu number. Decrease of metal ion content with the addition of anions resulted in decreased cfu number.
Since anions either reduced cfu number or did not affect it, we performed a second experiment, which detected significant impact of anion content, especially on archaea (Fig. 8).

Fig. 8. Results of experiment 2 on the study of the impact of anions on microbial growth for three strains (H. saccharovorum Н3а, Halorubrum sp. H7a, Halomonas sp. Н12b).
For the bacterium Halomonas sp. Н12b, cfu number slightly decreased with decreased concentrations of borates and nitrates, and increased with elimination of carbonates and phosphates from the medium.
For the archaea H. saccharovorum Н3а, cfu number decreased with elimination of carbonates and increased with elimination of nitrates and phosphates and decreased borate content; the effect of nitrate exclusion was the most pronounced.
We detected dramatic decrease of cfu number with carbonate elimination for Halorubrum sp. Н7а. This strain was more sensitive to changes in carbonate content than H. saccharovorum Н3а. Exclusion of nitrates and phosphates and decreased borate content resulted in several fold cfu increase for Halorubrum sp. Н7а.
Utilization of organic substrates by the studied strains
The studied strains failed to grow on Simmons citrate and amino acids (lysin, ornithin, arginin), as well as malonate (a salt of carboxylic acid). They were also incapable of enzymatic release of sulphur from sulphur containing amino acids or inorganic compounds (negative H2S test).
All strains produced urease, and thus could hydrolyse urea to ammonia. All strains could employ compounds of the following classes: polyols (inositol, adonitol, dultsitol, mannitol, sorbitol), trisaccharide (raffinose), disaccharides (cellobiose, sucrose, trehalose, melibiose) and monosaccharides (rhamnose, glucose).
Variation was observed only for β-galactosidase and esculin (Table 5). All archaeal strains were able to hydrolyse esculin (natural glucose ether), whereas bacterial strains were unable to degrade it. Four archaeal strain belonging to the genus Halorubrum possessed β-galactosidase activity, i.e. they were capable of cleaving lactose into glucose and galactose, which is also true for other representatives of this genus (Laye et al., Reference Laye, Karan, Kim, Pecher, DasSarma and DasSarma2017).
Table 5. Growth of the studied strains on various substrates

Discussion
In this study we used microorganisms from little studied and unique region in the Altai district to estimate the capability of terrestrial bacteria and archaea to survive at low temperatures and high concentration of salts and metals, similar to the Martian environment.
Resistance to freeze–thaw and desiccation cycles is a factor that would define the ability of terrestrial organisms to survive on Mars. Crisler et al. (Reference Crisler, Newville, Chen, Clark and Schneegurt2012) demonstrated that the number of surviving halotolerant bacteria is inversely proportional to the number of freeze–thaw cycles. The reduction in the number of viable bacteria was nearly linear with increasing freeze–thaw cycles. Our experiments corroborated these findings. However, this statement is still preliminary because it is based on analysis of only two freeze–thaw cycles while Crisler et al. (Reference Crisler, Newville, Chen, Clark and Schneegurt2012) performed 15 freeze–thaw cycles.
Second freezing is known to reduce cfu number relative to the first one. Mancinelli et al. (Reference Mancinelli, Fahlen, Landheim and Klovstad2004) demonstrated that non-halophilic Escherichia coli and Pseudomonas fluorescens failed to survive drying or freezing, while obligate halophiles could stand desiccation at 22 °C, as well as ten freeze–thaw cycles at −20 and −80 °C. In the study of de la Vega et al. (Reference De la Vega, Rettberg and Reitz2007) it was found that dehydrated Deinococcus radiodurans could survive a 7 day exposure to the Martian environment: temperature ranging from +14 to −63 °C under an atmosphere similar to that on Mars. It is also known that archaea of various morphologies demonstrate varying responses to extreme conditions resembling those on Mars; e.g. halococcal archaea proved to be more resilient than rod-shaped halobacteria (Stan-Lotter et al., Reference Stan-Lotter, Radax, Gruber, Legat, Pfaffenhuemer, Wieland, Leuko, Weidler, Kömle and Kargl2003). It is also known that microorganisms living in cryopegs are simultaneously halophilic and psychrophilic and remain metabolically active under such extreme conditions (Gilichinsky et al., Reference Gilichinsky, Rivkina, Bakermans, Shcherbakova, Petrovskaya, Ozerskaya, Ivanushkina, Kochkina, Laurinavichuis, Pecheritsina, Fattakhova and Tiedje2005). A strain of halophilic archaea isolated from the same district of the Altai region as those studied by us demonstrated a survival rate of 25% at −80 °C for wet samples (Peeters et al., Reference Peeters, Vos, Ten Kate, Selch, Van Sluis, Sorokin, Muijzer, Stan-Lotter, Van Loosdrecht and Ehrenfreund2010).
It is known that the Antarctic H. lacusprofundi has higher survival ability than the mesophilic Halobacterium sp. NRC-1 (DasSarma et al., Reference DasSarma, Laye, Harvey, Reid, Shultz, Yarborough, Lamb, Koske-Phillips, Herbst, Molina, Grah, Phillips and DasSarma2017). This study also found that most of the genes in the genome of the Antarctic species are significantly regulated at cold temperatures, which likely results in its better adaptation to the cold conditions in its natural environment and in the stratosphere (DasSarma et al., Reference DasSarma, Laye, Harvey, Reid, Shultz, Yarborough, Lamb, Koske-Phillips, Herbst, Molina, Grah, Phillips and DasSarma2017). The Antarctic H. lacusprofundi harbours a model polyextremophilic β-galactosidase that functions under cold, hypersaline conditions (Karan et al., Reference Karan, Capes, DasSarma and DasSarma2013; Laye et al., Reference Laye, Karan, Kim, Pecher, DasSarma and DasSarma2017; Laye and DasSarma, Reference Laye and DasSarma2018). A polyextremophilic β-galactosidase enzyme of H. lacusprofundi was studied in detail as a model enzyme for its evolution to extreme conditions (Karan et al., Reference Karan, Capes, DasSarma and DasSarma2013).
Thus, extensive studies of adaptive reserves of halophilic and psychrophilic bacteria and archaea suggest that certain terrestrial microorganisms are able to survive and grow under conditions close to those found on Mars. Some microbes from the Altai region may belong to such organisms. The strains isolated and studied by us demonstrated different responses to freeze–thawing at various NaCl concentrations. Thus, the studied bacteria grew and survived freeze–thawing at a wide range of NaCl concentrations. Most strains failed to survive freezing at 50 and 300 g l−1 NaCl. Archaea were able to survive freezing at NaCl concentrations over 200 g l−1. We found that larger cells demonstrated higher cfu number after freezing. Two freeze–thaw cycles at −70 °C resulted in an increase in cfu number. Analogous observations were made on cryopeg microorganisms and were explained by the fact that they could not only survive but also multiply under these conditions (Gilichinsky et al., Reference Gilichinsky, Soina and Petrova1993; Gilichinsky Reference Gilichinsky, Horneck and Baumstark-Khan2002). However, in our case this increase is more plausibly explained by disintegration of cellular aggregates after freezing (Golovach and Groma, Reference Golovach and Groma2013). This is indirectly supported by the fact that increased cfu number was observed only for archaea, which formed mucous colonies and biofilms (Liao et al., Reference Liao, Williams, Ye, Charlesworth, Burns, Poljak, Raftery and Cavicchioli2016), and not in solitary bacteria used in this study. It should be noted that such process could potentially increase the chances of archaea for distribution in the Solar System.
The ratio of survival fractions at −70 and −18 °C, the S(−70)/S(−18) value, can be used to study the effects of temperatures between −18 and −70 °C on survival. For the studied strains, survival fractions at −18 and −70 °C were comparable if survival fraction at −70 °C exceeded 80% (Fig. 9). However, S(−70)/S(−18) value increases with increasing survival fraction at −70 °C if S(−70) value was less than 80% (Fig. 9). It means that negative action of temperatures lower than −18 °C is stronger for strains which can only partly survive at −70 °C.

Fig. 9. The ratio of S(−18) and S(−70) values as function of S(−70) value. S(−18) and S(−70) are survival fractions after exposure to −18 and −70 °C, respectively. The NaCl content was 200 g l−1.
We should note that the rate of temperature changes at Viking 1 landing site during sunrise and sunset is about 20° h−1 (Murphy et al., Reference Murphy, Leovy and Tillman1990). This value is about 30 times lower than the typical heating and freezing rate in our experiments at similar temperatures (see Materials and methods section of this paper). As the survival rate after freezing increases with decreasing cooling and heating rates (Mazur, Reference Mazur1970; Tanghe et al., Reference Tanghe, Van Dijck, Colavizza and Thevelein2004), we expect that under the actual Martian conditions the studied strains would demonstrate a higher survival rate.
The r 2 correlation coefficient between survival fractions after freeze–thaw cycles (cycles 1 and 2) and cfu number in the control sample was high (about 0.9) (Fig. 10). It means that the survival fraction increases in more favourable media. We suggested that the chemical composition of lakes of the Altai region is ideal for microbes living in these lakes. For this reason we compared the chemical composition of the Altai lakes and the base solution in order to increase the growth rate, which is important, because increasing cfu of the studied strains will lead to increasing of survival fraction after action of low temperatures. It means that strains in ideal solutions will be survive better after action of low temperatures in comparison with that in non-ideal solutions.

Fig. 10. Dependence of the S(−18) value (survival fraction after cooling to −18 °C) on cfu in the control samples. The numbers 50, 100, 200 and 300 denote the NaCl content in the studied media (in g l−1).
Physicochemical parameters, primarily temperature, рН, Eh and chemical composition, define microorganism growth. However, we still lack information on the impact of various parameters on microorganism survival in extreme environments. Moreover, relatively little is known on how minor fluctuations of environmental conditions affect the microbial reproduction rate (Harrison et al., Reference Harrison, Hallsworth and Cockell2015). Thus, increased dataset about the impact of various geochemical parameters on microorganisms will allow evaluating the borders of life in the Solar System.
Mathematical modelling suggests that saline liquids with high concentrations Na+, K+, Mg2+, Fe2+, Cl– and SO42– can exist on the Martian surface (Tosca et al., Reference Tosca, McLennan, Lamb and Grotzinger2011).
Comparison of Mg2+ and Na+ content in the Altai lakes and studied media showed that in the majority of our experiments Mg2+ content was lower than in the Altai lakes, while Na+ content was comparable with that in the Altai lakes (Figs. 11 and 12). In the majority of our experiments SO42− content was lower than in the Altai lakes, while Cl− content was comparable with that in the Altai lakes (Fig. 12). We should note that in our experiments we were unable to cover the whole range of Na+, SO42− and Cl− content in the studied Altai lakes (see Figs. 11 and 12). For example, in studied lakes maximal content of Na+, SO42− and Cl− reaches 110, 150 and 180 g l−1, respectively, while in our experiments there values are 80, 80 and 130 g l−1, respectively (see also Bryanskaya et al., Reference Bryanskaya, Berezhnoy, Rozanov, Peltek and Pavlov2013).

Fig. 11. Comparison of the chemical composition of saline lakes of the Altai region, media used in our experiments, and those used by Stan-Lotter et al. (Reference Stan-Lotter, Radax, Gruber, Legat, Pfaffenhuemer, Wieland, Leuko, Weidler, Kömle and Kargl2003), Crisler et al. (Reference Crisler, Newville, Chen, Clark and Schneegurt2012) on Mg2+–Na+ axes.
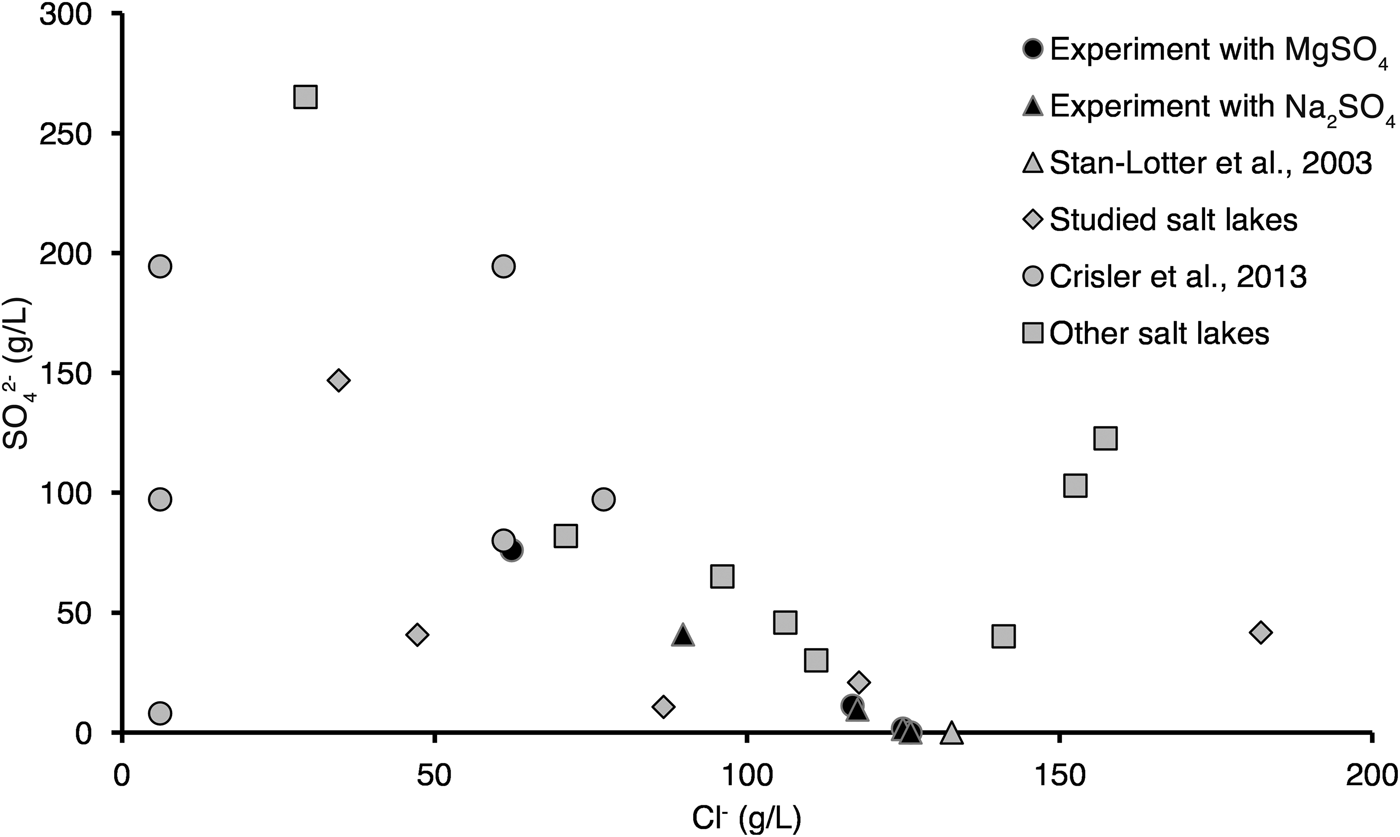
Fig. 12. Comparison of the chemical composition of the Altai saline lakes, media used in our experiments and in those of Stan-Lotter et al. (Reference Stan-Lotter, Radax, Gruber, Legat, Pfaffenhuemer, Wieland, Leuko, Weidler, Kömle and Kargl2003) and Crisler et al. (Reference Crisler, Newville, Chen, Clark and Schneegurt2012) on Mg2+–Na+ (top) and SO42−–Cl− axes (bottom). Chemical composition of other salt lakes was taken from Lebedeva et al. (Reference Lebedeva (Verba), Lopukhina and Kalinina2008), and studied lakes from Bryanskaya et al. (Reference Bryanskaya, Malup, Lazareva, Taran, Rozanov, Efimov and Peltek2016).
The comparison of the elemental composition of Altai salt lakes and the basic solution shows that many substances including anions such as NO3−, HCO3− and HPO42−, were absent in the base solution, while the content of other ions such as WO4−, Fe2+, Co2+ and Mn2+ was enriched in comparison with salt lakes (Table 4). For this reason growth of selected strains at different content of cations of metals and anions (phosphates, nitrates, carbonates and borates) was studied. We found that the studied strains reacted similarly to changes in medium content: decrease in metal content resulted in an increase in cfu number. It is noteworthy that 100-fold decrease in metal content did not lead to further increases in cfu number, which can be explained by the fact that metal content in the base medium was much more than in the source lakes, so decreasing metal concentrations makes medium closer to the native environment for the strains.
We found that the bacterium Halomonas sp. H12b was highly tolerant to changes in medium content, while the archaea H. saccharovorum H3a and Halorubrum sp. H7a were highly sensitive to such changes. Growth of all studied strains depended on carbonate content but was independent on nitrates, borates and phosphates in the studied concentrations. This could be possibly explained by the fact that chloride and carbonate anions are interchangeable (Boltyanskaya et al., Reference Boltyanskaya, Antipov, Kolganova, Lysenko, Kostrikina and Zhilina2004), and the studied archaea are halophilic.
All studied anions and cations play an important role in the metabolism of microorganisms, so their concentrations affect growth and survival (Rivadeneyra et al., Reference Rivadeneyra, Delgado, Ramos-Cormenzana and Delgado1998; Ventosa et al., Reference Ventosa, Nieto and Oren1998; Smirnov et al., Reference Smirnov, Suzina, Chudinova, Kulakovskaya and Kulaev2005; Ahmed et al., Reference Ahmed, Yokota and Fujiwara2007; Nelson and Mele, Reference Nelson and Mele2007; Antony et al., Reference Antony, Sujith, Fernandes, Verma, Khedekar and Bharathi2011; Kraft et al., Reference Kraft, Strous and Tegetmeyer2011; Harrison et al., Reference Harrison, Hallsworth and Cockell2015).
Search for organic compounds on Mars has a long history. The main goal of the Viking missions was to estimate the possibility of life on Mars. Organic compounds (chloromethane CH3Cl and dichloromethane CH2Cl2) detected by Viking by thermal volatilization gas chromatograph mass spectrometry after heating Martian samples was attributed to terrestrial contamination in the instruments (Biemann et al., Reference Biemann, Oro, Toulmin, Orgel, Nier, Anderson, Flory, Diaz, Rushneck and Simmonds1977). The same explanation was applied to several chlorinated hydrocarbons detected by the SAM instrument on board of the Mars Science Laboratory in the Gale crater (Glavin et al., Reference Glavin, Freissinet, Miller, Eigenbrode, Brunner, Buch, Sutter, Archer, Atreya, Brinckerhoff, Cabane, Coll, Conrad, Coscia, Dworkin, Franz, Grotzinger, Leshin, Martin, McKay, Ming, Navarro-González, Pavlov, Steele, Summons, Szopa, Teinturier and Mahaffy2013). Recently, the concentrations of perchlorates and organic carbon at the Viking landing sites have been estimated at <0.1% and 0.7–6.5 ppm, respectively (Navarro-González et al., Reference Navarro-González, Vargas, De la Rosa, Raga and McKay2010). High concentrations of chlorides, perchlorates and soluble sulphates were proven to be present in Martian soils (Kounaves et al., Reference Kounaves, Hecht, Kapit, Quinn, Catling, Clark, Ming, Gospodinova, Hredzak, McElhoney and Shusterman2010; Taylor et al., Reference Taylor, Boynton, McLennan and Martel2010). High abundance of perchlorates (around 1%) was discovered at the Phoenix landing site (Hecht et al., Reference Hecht, Kounaves, Quinn, West, Young, Ming, Catling, Clark, Boynton, Hoffman, Deflores, Gospodinova, Kapit and Smith2009). High content of perchlorates and very low content of organic compounds make present-day Mars inhospitable for majority of terrestrial life forms. However, perchlorate resistant halophiles were found on Earth (Al Soudi et al., Reference Al Soudi, Farhat, Chen, Clark and Schneegurt2017; Matsubara et al., Reference Matsubara, Fujishima, Saltikov, Nakamura and Rothschild2017; Laye and DasSarma, Reference Laye and DasSarma2018). Let us note that early Mars was warmer and wetter than present-day Mars (Urata and Toon, Reference Urata and Toon2013), such conditions on early Mars were more hospitable for microbial life (Litchfield, Reference Litchfield1998; Schulze-Makuch et al., Reference Schulze-Makuch, Irwin, Lipps, LeMone, Dohm and Fairén2005).
Prebiotic compounds may be delivered to planets, including Mars, by low-speed impacts of comets and other volatile-rich celestial bodies (Pierazzo and Chyba, Reference Pierazzo and Chyba1999). Comets and carbonaceous chondrites are the most primitive bodies of the Solar System, because these objects were never heated to high temperatures and retained their initial organic material. The average hydrogen and carbon content by the number of atoms in carbonaceous chondrites is 30 and 4.4%, respectively (Lodders, Reference Lodders2003), while for the Halley comet these values are as high as 48 and 14% (Delsemme, Reference Delsemme1988).
However, not all carbon is in biologically accessible form. A significant fraction of meteoritic organics is represented by acylated and unacylated polycyclic aromatic hydrocarbons (Elsila et al., Reference Elsila, De Leon, Buseck and Zare2005), as well as by various aliphatic compounds (Garvie and Buseck, Reference Garvie and Buseck2007). The content of organic compounds containing carboxylic groups is only about 10% in CI chondrites (Garvie and Buseck, Reference Garvie and Buseck2007).
The majority of compounds used in our experiments are too complex to be detected in meteorites. However, amino acids and sugars were detected in stony meteorites in small amounts: e.g. sugar content in CI chondrites ranges from 5 to 26 ppm, and mannose and glucose are the most abundant meteoritic sugars (Kaplan et al., Reference Kaplan, Degens and Reuter1963). Later, various polyols such as sugars, sugar alcohols and sugar acids, were identified in the well-studied Murchison meteorite by Cooper et al. (Reference Cooper, Kimmich, Belisle, Sarinana, Brabham and Garrel2001). Amino acids are present in greater concentrations, about 30–500 ppm, in CI chondrites (Kaplan et al., Reference Kaplan, Degens and Reuter1963), while the content of amino acids in CM2 chondrites is much smaller (Glavin et al., Reference Glavin, Dworkin, Aubrey, Botta, Doty, Martins and Bada2006).
Our study employed various substrates found on meteorites and other cosmic objects; we found that the studied strains could grow on polyhydric alcohols, tri-, di- and monosaccharides, but not such amino acids as lysin, ornithin and arginin, as well as sulphur-containing substances (see Table 5). Terrestrial extremophilic microbes can employ a wide variety of organic substrates; e.g. the psychrotolerant Antarctic anaerobe ISLP-3 can grow on D-glucose, D-ribose, D-fructose, D-arabinose, maltose, sucrose, D-trehalose, D-mannose, D-cellobiose, lactose, starch, chitin, triethylamine, N-acetylglucosamine and urea (Guisler et al., Reference Guisler, Pikuta, Townsend and Hoover2009).
Although it is known that ecologic parameters of the environment affect the growth rate and reaction to physicochemical parameters of cultivation (Reid et al., Reference Reid, Sparks, Lubow, McGrath, Livio, Valenti, Sowers, Shukla, MacAuley, Miller, Suvanasuthi, Belas, Colman, Robb, DasSarma, Müller, Coker, Cavicchioli, Chen and DasSarma2006; Vishnivetskaya et al., Reference Vishnivetskaya, Petrova, Urbance, Ponder, Moyer, Gilichinsky and Tiedje2006; DasSarma et al., Reference DasSarma, Laye, Harvey, Reid, Shultz, Yarborough, Lamb, Koske-Phillips, Herbst, Molina, Grah, Phillips and DasSarma2017), we found no correlation between environmental conditions in the source lakes and survival rates at varying temperature, salinity and the number of freeze–thaw cycles. Halorubrum strains isolated from different lakes (Halorubrum sp. Н4a, Halorubrum sp. Н11a, Halorubrum sp. Н13a) demonstrated similar survival rates, while those from a single lake (e.g. H. saccharovorum H3a and Halorubrum sp. H4a from the Burlinskoye lake) had different survival rates.
At the same time, survivability of bacteria and archaea varied, which could be caused by different strategies of adaptation to osmotic stress (Mancinelli et al., Reference Mancinelli, Fahlen, Landheim and Klovstad2004). Closely related strains H. saccharovorum H3a and Halorubrum sp. H7a isolated from different lakes had different survival rates. Similar observations were made by Peeters et al. (Reference Peeters, Vos, Ten Kate, Selch, Van Sluis, Sorokin, Muijzer, Stan-Lotter, Van Loosdrecht and Ehrenfreund2010).
Conclusions
Our studies on search and isolation of microorganisms as well as characterization of survival rate of microorganisms at high salt content, low temperatures, different concentrations of anions and cations and different organic substrates, are useful for deeper understanding of survival strategies of microorganisms on Earth. Based on our results we suggest that both halophilic archaea and halotolerant bacteria from saline lakes of the Altai region (the remnants of an ancient sea) may be considered as analogues of ancient Martian organisms, since they are able to withstand conditions that hypothetically existed with only slight decrease in viability in subsurface layers of the early Mars (low temperatures, salt solutions with a high content of NaCl, MgSO4, Na2SO4). We also found that the studied microorganisms can use organic substances found in meteorites. Based on these results, we believe that transfer of unicellular halophiles from Earth to Mars was possible, and, moreover, they could successfully survive and grow on early Mars. Adjusting our growth media to the chemical composition of the lakes from which the studied strains were isolated resulted in significant increase in survival and growth rates. Certain strains could survive several freeze–thaw cycles at −70 °C typical for Martian nights. In subsequent studies we will search for the optimal medium for further studies of the effects of low temperatures on growth and survival of Altai halophiles at various concentrations of anions and metal cations.
Acknowledgements
The reported study was funded by RFBR and Novosibirsk region according to the research project no. 17-44-540815, and by ICG SB RAS Budget Project no. 0324-2018-0017 ‘Genetic basis of biotechnology and bioinformatics’. This work was done at the Center for collective use ‘A collection of biotechnological microorganisms as a source of novel promising objects for biotechnology and bioengineering’ of Federal Research Center ‘Institute of Cytology and Genetics of the Siberian Branch of the RAS’.