Introduction
Invasive alien plants (IAPs) reduce biodiversity and the capacity of rangelands to support livestock production (Jones and McDermott, Reference Jones and McDermott2018). Amongst IAPs, the genus Acacia sensu stricto (formerly subgenus Phyllodineae) native to Australia is the most widespread with 24 out of 1000 species classified as invasive globally (Richardson et al., Reference Richardson, Le Roux and Wilson2015). In South Africa, black wattle (Acacia mearnsii) and silver wattle (A. dealbata) are the dominant IAPs covering about 10 million hectares of mesic areas land (Gwate et al., Reference Gwate, Mantel, Finca, Gibson, Munch and Palmer2016; Gouws and Shackleton, Reference Gouws and Shackleton2019). These species were introduced in South Africa from Australia for the commercial production of tannins and woodchips for export but became invasive (Chan et al., Reference Chan, Day, Feely, Thompson, Little and Norris2015). Control of A. mearnsii and A. dealbata species has, therefore, become a policy priority for the South African government (Richardson et al., Reference Richardson, Le Roux and Wilson2015; Shackleton et al., Reference Shackleton, Shackleton and Kull2018). Mechanical and chemical methods, which involve cutting the Acacias with chainsaws and applying herbicides on the cut stump are currently being used to prevent new establishments and reducing existing ones (Richardson et al., Reference Richardson, Le Roux and Wilson2015; Shackleton et al., Reference Shackleton, Shackleton and Kull2018). In the smallholder farming areas, the cut branches are used for fencing, firewood or charcoal (De Neergaard et al., Reference De Neergaard, Saarnak, Hill, Khanyile, Berzosa and Birch-Thomsen2005; Gouws and Shackleton, Reference Gouws and Shackleton2019) but leaves are left to decompose naturally.
Acacia trees have high rates of leaf-litter accumulation that is deposited in large quantities, which become waste and proliferate pests and diseases over time (Eyles et al., Reference Eyles, Beadle, Barry, Francis, Glen and Mohammed2008). These leaves could be beneficial for improving the production and quality of animal-sourced foods as they contain moderate contents of crude protein (CP, 130–175 g/kg DM; Salem, Reference Salem2005), high contents of neutral detergent fibre (NDF, 180–457 g/kg; De Neergaard et al., Reference De Neergaard, Saarnak, Hill, Khanyile, Berzosa and Birch-Thomsen2005; Giridhar et al., Reference Giridhar, Prabhu, Singh, Nagabhushan, Thirumalesh, Rajeshwari and Umashankar2018) and proanthocyanidins (up to 150 g/kg DM; Degen et al., Reference Degen, Kam and Makkar2000; Xiong et al., Reference Xiong, Grace, Esposito, Wang and Lila2016) that have nutritional, helminth suppressant, anti-bloat, antioxidant and antimicrobial properties (Sottie et al., Reference Sottie, Acharya, McAllister, Thomas, Wang and Iwaasa2014; Yoshihara et al., Reference Yoshihara, Minho, Cardim, Tabacow and Yamamura2014; Koenig et al., Reference Koenig, Beauchemin and Mcginn2018; Lima et al., Reference Lima, Crouzoulon, Sanches, Zabré, Kabore, Niderkorn, Hoste, Talamini Do Amarante, Martins Costa-Júnior, Luiz Abdalla and Louvandini2019). In this regard, the valorisation of Acacia leaves presents an economic incentive for the sustainable management of IAPs and opportunities to unlock new value chains for the Acacia and animal feed industries to progress towards a circular bioeconomy.
The utilisation of Acacia leaves as livestock feed is dependent upon their nutritional composition and bioactive phytochemical profile, which are largely influenced by biotic and abiotic environmental factors, particularity plant species and seasons, respectively (Mueller-Harvey et al., Reference Mueller-Harvey, Bee, Dohme-Meier, Hoste, Karonen, Kölliker, Lüscher, Niderkorn, Pellikaan, Salminen, Skøt, Smith, Thamsborg, Totterdell, Wilkinson, Williams, Azuhnwi, Baert, Brinkhaus, Copani, Desrues, Drake, Engström, Fryganas, Girard, Huyen, Kempf, Malisch, Mora-Ortiz, Quijada, Ramsay, Ropiak and Waghorn2019). Intra- and inter-species chemical variability and diversity is attributable to the interactive effects of plant genes, ontogeny, phenology and phenotypic plasticity (Moore et al., Reference Moore, Andrew, Külheim and Foley2014; Hasan et al., Reference Hasan, Rusman, Khaldun, Ardana, Mudatsir and Fansuri2020). The phenotypic plasticity of plants is largely influenced by seasonality (Chuine and Régnière, Reference Chuine and Régnière2017). Seasonal changes result in annual variations in day length, temperature, light, radiation, humidity, precipitation and nutrient supply, which subsequently influence plant chemical profiles (Williams et al., Reference Williams, Ragland, Betini, Buckley, Cheviron, Donohue, Hereford, Humphries, Lisovski, Marshall, Schmidt, Sheldon, Varpe and Visser2017; Choudhary et al., Reference Choudhary, Thakur, Jaitak and Bhardwaj2019). However, there is a dearth of information regarding the effects of season on nutritional, phytochemical and antioxidant profiles of A. mearnsii and A. dealbata leaves. The information is crucial in unmasking the potential of Acacia leaves as nutraceuticals and preservatives for enhancing ruminant production and product quality, respectively. The objective of the current study was, therefore, to evaluate the effects of season on the nutrient, phytochemical and antioxidant profiles of A. mearnsii and A. dealbata leaves.
Materials and methods
Study site, collection and preparation of Acacia leaf-meals
Acacia mearnsii and A. dealbata leaves were harvested during the hot-dry (i.e. February) and cool-wet (i.e. June) seasons in two locations (33°52′27″ 18°58′33″E; 33°55′31.8″S 18°52′45.3″E, respectively) in the Mediterranean region of Pniel, South Africa. These two locations are in the same agroecological zone with an annual temperature ranging from 6 to 30°C and receive rainfall between 250 and 350 mm per annum. A systematic sampling technique was used to sample Acacia trees for leaf harvesting. Two 100 m transect lines were set diagonally across each other in a one-hectare plot and held in place by metal pins. Along each transect line, four 5 × 5 m2 quadrants were placed at 20 m intervals using a measuring tape. In each quadrant, leaves were harvested from four trees with a breast height diameter between 6 and 8 cm by pruning six lower branches from each tree. The harvested leaves from each quadrant (n = 8/species) were placed in brown khaki bags and transported to the Department of Animal Sciences at Stellenbosch University. Exactly, 2 kg of fresh leaves per sample were oven-dried at 50°C for 72 h according to Makkar (Reference Makkar2003) and ground using a Wiley mill (Model 4, Thomas Scientific, Swedesboro, NJ, USA) with a 1 mm sieve and stored in pre-labelled airtight containers at 4°C pending analyses.
Proximate and fibre analysis
The AOAC (2002) procedures were used to determine the dry matter (DM), ash and ether extract (EE) contents of the samples. The Dumas method with a macro-Nitrogen analyser (LECO® FP528, LECO Corporation, Miami, USA) was used to determine total nitrogen content and multiplied by a 6.25 factor to calculate the crude protein (CP) content. Starch was analysed using a commercial assay (Total Starch Megazyme kit KTSTA, Megazyme International Ireland Ltd., Wicklow, Ireland), following methodology by Hall (Reference Hall2009). Neutral detergent fibre (aNDFom) was analysed according to Mertens (Reference Mertens2002), using sodium sulphite and alpha-amylase. Acid detergent fibre (ADFom) was determined according to AOAC (2002). Lignin (sa) was determined by solubilization of cellulose with sulphuric acid according to Raffrenato and Van Amburgh (Reference Raffrenato and Van Amburgh2011). Neutral detergent fibre, ADFom and lignin (sa) were expressed exclusive of ash content. All analyses were performed in triplicate. The non-fibrous carbohydrates (NFC) were determined using the following formula:

Neutral detergent soluble fibre (NDSF) was computed by subtracting aNDFom (g/kg) from 1000 g/kg. Pectin and sugars were calculated according to López et al. (Reference López, Estellés, Moya and Fernández2014) by subtracting the content of starch (g/kg DM) from NFC (g/kg) content.
In vitro digestibility analysis
Two rumen-cannulated Holstein dairy cows were used as rumen content donors for the in vitro aNDFom digestibility (ivNDFd). The animals were grazing on Kikiyu pasture, and the rumen fluid was collected at 08:00 before the animals were let out for grazing. The rumen fluid was immediately transported to the laboratory in a pre-warmed insulated thermos flask. The rumen fluid was filtered through four layers of cheesecloth, 100 μm mesh and glass wool prior to inoculation. About ~0.5 g ground sample of A. mearnsii and A. dealbata leaf-meals in duplicates were weighed into 125 ml Erlenmeyer flask, with the addition of 40 ml of Van Soest buffer according to Raffrenato et al. (Reference Raffrenato, Ross and Van Amburgh2018). The flasks were placed in a heated (39.5°C) shaking water bath under carbon dioxide positive pressure to ensure an anaerobic environment, thereafter, 10 ml of rumen fluid was added.
In vitro aNDFom digestibility was measured at 24 and 48 h. After incubation, the undigested matter was analysed for aNDFom content by filtering the boiled samples through Gooch sintered glass crucible (40–60 μm) crucibles with a Whatman glass microfiber filter (934-AH®, GE Healthcare, Pittsburgh, PA, USA), which minimises particle loss and increase recovery. The difference between the undigested aNDFom after boiling and the aNDFom content of the leaves was used to calculate the digestibility coefficient of ivNDFd on a DM basis. Each sample within treatment was analysed in duplicate, and the analysis was performed in three runs.
Amino acid analysis
Waters Acquity Ultra Performance Liquid Chromatograph (UPLC) fitted with a photo-diode array detector (UPLC-PDA) was used for separation and detection of amino acids (AA) using AccQ Fluor reagent Kit, Waters (En Yvelines Cedex, France). Briefly, 0.1 g grounded dried leaf-meals of A. mearnsii and A. dealbata was used to determine AA composition using 0.5 ml of 6 M hydrochloric acid for extraction and L-Norvaline as an internal standard. In the vials, 10 μl of the standard and sample was added, with 70 μl buffer solution (0.2 M borate buffer) and 20 μl of derivatisation reagent (2 mg/ml 6-aminoquinolyl-N-hydroxysuccinimidyl carbamate). The vials were incubated at 55°C for 10 min to build stable derivatives. A volume of 1 μl of standard/sample solution was injected into the mobile phase onto the Waters UltraTag C18 column (2.1 × 50 mm × 1.7 μm) at 60°C. The amino acids were quantified against a 16 amino acid standard (AAS18-5ml, Sigma-Aldrich, USA). During the development of the chromatographic method, individual amino acids standards were prepared to identify the unique retention time of each amino acid. After derivatization of the calibration standards, they were analysed using the Waters AQC UPLC chromatographic method. The gradient of the method was adjusted until baseline separation of all 16 amino acids, the AMQ peak from the derivatizing agent and the L-Norvaline internal standard peak was achieved. Analytes eluting off the column were detected by the PDA detector, with each AA coming off the column at a unique retention time. Calibration curve was plotted, and AA concentration quantified in MassLynx V4.1 2011 software (Waters, Milford, USA), using peak areas and retention times. The analyses were performed in triplicate per sample.
Mineral content analysis
Minerals were quantified using the inductively coupled plasma-mass spectroscopy (ICPMS) and –atomic emission spectrometry (ICPAES) for micro, and macro minerals, respectively, according to Sah and Miller (Reference Sah and Miller1992). The samples were digested using CEM MARS microwave digester. For ICPMS digested solid samples were introduced into the instrument via autosampler by a peristaltic pump. Small droplets pass through to the plasma, which produces ions that are directed into the MS and extracted from the interface region. An ion detector converts the ions into an electrical signal. This signal is expressed as counts using Masshunter software for calculating results using a calibration acceptance criterion of R 2 > 0.9995. For ICPAES, the ions excite in the plasma and emit characteristic light, measured by the Echelle optical design and charge injection device solid-state detector to provide elemental analysis. The instrument was controlled, and data were processed by iTEVA software using a calibration acceptance criterion of R 2 > 0.9995. The analyses were performed in triplicate.
Fatty acids analysis
Following the procedures of Sukhija and Palmquist (Reference Sukhija and Palmquist1988), individual and total lipids for A. mearnsii and A. dealbata leaf-meals were determined in triplicate. Briefly, 2.5 ml of n-hexane was used to extract 0.5 g dried samples with 100 μl of 0.1 ml/l of heptadecanoic acid as an internal standard. Thereafter, 1 ml of 20% (v/v) sulphuric acid in methanol was added for the derivatisation step. The samples were then vortexed and incubated at 70°C for 60 min in a water bath. After cooling at room temperature, 2 ml of 20% (w/v) sodium chloride was added and samples vortexed. The top layer was transferred using a glass Pasteur pipette into fatty acid methyl esters (FAMEs) vials. The FAMEs were analysed using a gas chromatograph (6890 N, Agilent Technologies) coupled to a flame ionisation detector. Separation of the FAMEs was performed on a polar RT-2560 (100 m length × 0.25 mm internal diameter × 0.20 μm film thickness) (Restek, USA) capillary column. Helium was used as the carrier gas at a flow rate of 1 ml/min. The injector temperature was maintained at 250°C and 1 μl of the sample was injected in a 5:1 split ratio. The oven temperature was programmed at 50°C for 2 min and ramped up to 180°C at a rate of 25°C/min and held for 5 min and finally ramped up to 250°C at a rate of 3°C for 2 min. The peak areas and retention times relative to the Supelco™ 37 FAME mix, (Supelco, USA) were used to identify the FAME. The content of fatty acids (FA) was expressed as g/100 g of total FAME.
Polyphenol extraction
About 500 g of dried A. mearnsii and A. dealbata leaves were ground to pass a 2 mm sieve on a Wiley mill (Model 4, Thomas Scientific, Swedesboro, NJ, USA), thereafter, 100 g of the ground sample was further ground to pass a 0.5 mm sieve. For extraction, 20 ml of 80% aqueous methanol (v/v) was added to 2 g of the ground sample and placed in an ultrasonic water bath for 20 min and then centrifuged for 20 min set at 10 000 × g at 4°C. The supernatant was collected, and the sample was re-extracted using 20 ml of 80% aqueous methanol (v/v). The supernatants were mixed and stored at −80°C pending for analysis. Before analysis, the supernatants were diluted by 63.7% for all analyses besides simple phenolics analysis which was diluted at 21.2%. All polyphenols were analysed in triplicate.
Polyphenol analysis
Individual phenolic compounds were identified based on methods described by Tsugawa et al. (Reference Tsugawa, Cajka, Kind, Ma, Higgins, Ikeda, Kanazawa, Vandergheynst, Fiehn and Arita2015) and Lai et al. (Reference Lai, Tsugawa, Wohlgemuth, Mehta, Mueller, Zheng, Ogiwara, Meissen, Showalter, Takeuchi, Kind, Beal, Arita and Fiehn2018). A waters synapt G2 quadrupole time-of-flight (QTOF) mass spectrometer (MS) connected to a UPLC (Waters, Milford, MA, USA) was used for greater-resolution UPLC-MS analysis. Column eluate first passed through a photodiode array (PDA) detector before going to the MS, allowing simultaneous collection of UV and MS spectra. Electrospray ionisation was used in negative mode with a cone voltage of 15 V, desolvation temperature of 275°C, desolvation gas at 650 l/h and the rest of the MS settings optimised for best resolution and sensitivity. Data were acquired by scanning from 150 to 1500 m/z in resolution mode as well as in MSE mode. In MSE mode two channels of MS data were acquired, one at a low collision energy (4 V) and the second using a collision energy ramp (40–100 V) to obtain fragmentation data. Leucine enkaphalin was used as lock mass (reference mass) for accurate mass determination and the instrument was calibrated with sodium formate. Separation was achieved on a waters HSS T3, 2.1 × 100 mm, 1.7 μm column. An injection volume of 2 μl was used and the mobile phase consisted of 0.1% formic acid (solvent A) and acetonitrile containing 0.1% formic acid as solvent B. Data were processed using MSDIAL and MSFINDER (RIKEN Center for Sustainable Resource Science: Metabolome Informatics Research Team, Kanagawa, Japan).
The Folin-Ciocalteu colorimetric method as described by Makkar (Reference Makkar2003) was used for the determination of total phenolics and tannin contents using ultraviolet (UV)-visible spectrophotometer (Thermo Scientific Technologies, Madison, WI, USA) and absorbance was read at 725 nm. The tannin content was calculated as follows; total phenolics without polyvinylpyrrolidone minus total phenolics with polyvinylpyrrolidone addition. A calibration curve was obtained using gallic acid and samples were analysed in triplicate. The results were expressed on a gram gallic acid equivalent (GAE)/kg DM. Proanthocyanidins were determined following the procedure of Porter et al. (Reference Porter, Hrstich and Chan1986) and the results were expressed as g/kg DM leucocyanidin equivalent.
The total contents of flavonoids were determined following the procedure by Yang et al. (Reference Yang, Martinson and Liu2009). Briefly, a 0.25 ml aliquot of extracts (63.7% dilution) was mixed with 1.25 ml of deionised water and added to 0.075 ml of 5% sodium nitrite solution. The solution was incubated for 5 min then 0.15 ml of 10% aluminium chloride was added and incubated for 5 min. Thereafter, 0.5 ml of 1 M sodium hydroxide was added to stop the reaction and 0.775 ml of deionised water was added and read at 510 nm using a UV-visible spectrophotometer. The total flavonoids content was calculated using a catechin standard curve and expressed as g of catechin equivalents (CE)/kg extract DM.
Antioxidant activity analysis
The 2,2-diphenyl-1-picrylhydrazyl (DPPH) radical scavenging activity was performed in triplicate following methodology by Tolic et al. (Reference Tolic, Jurcevic, Krbavcic, Markovic, Vahcic, Juvcevic, Krbavcic, Markovic, Vahcic, Jurcevic, Krbavcic, Markovic and Vahcic2015). A 20 μl aliquot of sample extracts was diluted in 1 ml of 80% methanol and mixed with an equal volume of 0.1 mm DPPH reagent and incubated in the dark for 30 min and read at 517 nm absorbance using a UV-visible spectrophotometer. Methanol was used as a negative control and L-ascorbic acid as a positive control. The results were expressed as % radical scavenging activity.
The ferric reducing antioxidant power (FRAP) assay was conducted in triplicate as outlined by Benzie and Strain (Reference Benzie and Strain1996). Aliquots of freshly prepared reagent containing 25 ml of 300 mm acetate buffer (pH 3.6), 2.5 ml of 20 mm of iron (III) heptahydrate and 2.5 ml of 10 mm 2,4,6-Tris(2-pyridyl)-s-triazine (TPTZ) solution was mixed with plant extracts before incubation for 30 min in the dark. The method uses low pH to reduce the Fe3+-2,4,6-tris(pyridyl)-s-triazine (TPTZ) complex to the ferrous form. This reduction is monitored by measuring the absorbance change at 595 nm. The standard curve was prepared using Trolox and the FRAP values were reported as μmol Trolox equivalent per g DM.
A 15-Lipoxygenase (15-LOX) inhibition assay was conducted according to Waslidge and Hayes (Reference Waslidge and Hayes1995). Dimethyl sulfoxide (DMSO) was used as a negative control and 15-LOX as a positive control. The 100% initial activity (IA) wells were prepared by adding 50 μl of LOX and 20 μl of 10% DMSO. Sample wells (inhibitor) contained 50 μl of LOX and 20 μl of extract. The reaction was initiated by adding 50 μl of linoleic acid (140 μm) to all the wells and incubated for 10 min and 100 μl of 15-LOX as added to terminate the reaction. The Fe3+-dye complex was allowed to develop for 30 min at 25°C and the absorbance was read at 570 nm on a UV-visible spectrophotometer and the result expressed % inhibition of lipoxygenase activity. The per cent lipoxygenase inhibition for each extract was determined as follows:
Inhibition = [1–IA − Inhibitor)/IA] × 100. To quantitatively evaluate the overall phytochemical quality, the antioxidant potency composite (APC) index was calculated based on antioxidant index score = [(sample score/best score) × 100], averaged for all four tests for each extract.
Statistical analysis
The data were analysed as a completely randomized design with a 2 (seasons) × 2 (Acacia species) factorial arrangement of treatments using the generalized linear model procedure of SAS v.9.4 (SAS Institute Inc., Cary, NC, USA). The total number of observations for proximate, AA, FA, DPPH, FRAP, LOX, total flavonoids, total phenols, total tannins and proanthocyanidins analyses were 96 (2 Acacia species × 2 seasons × 8 samples × 3 replicates) and for minerals and phenolic compounds were 48 (2 Acacia species × 2 seasons × 4 samples × 3 replicates). For ivNDFd the total number of observations was 192 (2 Acacia species × 2 seasons × 8 samples × 2 replicates × 3 runs). After averaging the replications and/or runs, 32 (proximate, ivNDFd, AA, FA, DPPH, FRAP, 15-LOX, total flavonoids, total phenols, total tannins and proanthocyanidins), and 16 (minerals and phenolic compounds) observations were subjected to analysis of variance using the following statistical model: Yijk = μ + Ai + Bj + A × Bij + ɛ ijk where: Yijk = variable response of the kth observation in ith species and jth season, μ = overall mean, Ai = effect of ith species (i = A. mearnsii and A. dealbata), Bj = effect of the jth season (j = hot-dry and cool-wet), A × Bij = interaction of ith species and jth season, ɛ ijk = residual error. The treatment means were generated using the least-square means option. Tukey's test was applied for LSMEANS separation. Results were considered significantly different at P < 0.05.
Results
Chemical composition
Acacia species and season interaction had a significant effect on all the analysed proximate components (Table 1). Contents of DM and lignin (sa) in the leaf-meals were in the following order: A. dealbata × hot-dry-season > A. mearnsii × hot-dry-season > A. mearnsii × cool-wet-season > A. dealbata × cool-wet-season (P < 0.05). The ash content was greatest in A. dealbata leaf-meal in the hot-dry-season, lowest in A. dealbata × cool-wet-season leaf-meal and intermediate in A. mearnsii leaf-meals irrespective of the season (P < 0.05). The CP and NDSF contents of the leaf meals were in the order of A. mearnsii × hot-dry-season > A. dealbata × hot-dry-season > A. dealbata × cool-wet-season > A. mearnsii × cool-wet-season (P < 0.05). Starch content was greatest in A. dealbata × cool-wet-season followed by A. dealbata × hot-dry-season, A. mearnsii × cool-wet-season and A. mearnsii × hot-dry-season (P < 0.05). Ether extract was lowest in leaf-meal of A. dealbata in the cool-wet-season, and highest in A. mearnsii × hot-dry-season (P < 0.05). The aNDFom content in Acacia leaf-meals was in the order of A. mearnsii × cool-wet-season > A. dealbata × cool-wet-season > A. dealbata × hot-dry-season > A. mearnsii × hot-dry-season (P < 0.05). The ADFom and NFC contents of Acacia leaf-meals were lowest in A. mearnsii × hot-dry-season, greatest in A. dealbata × cool-wet-season and intermediate in both A. dealbata × hot-dry-season and A. mearnsii × cool-wet-season leaf-meals (P < 0.05). Pectin and sugars were greatest in the A. mearnsii × hot-dry-season leaf-meal, intermediate in the A. mearnsii × cool-wet-season and lowest in the A. dealbata leaf-meals regardless of season (P < 0.05).
Table 1. Least square means of the proximate and fibre contents, and in vitro digestibility of A. mearnsii and A. dealbata leaf-meals in the hot-dry and cool-wet seasons

s.e.m, standard error of means; aNDFom, neutral detergent fibre exclusive of ash.
In vitro neutral detergent fibre digestibility
For 24 and 48 h ivNDFd, significant effects were observed for species, season and their interaction (Table 1). Acacia mearnsii leaf-meal harvested in the hot-dry-season had the greatest coefficient ivNDFd at 24 and 48 h, with cool-wet-season leaf-meals having intermediate values regardless of species while lowest for A. dealbata leaf-meal harvested in the hot-dry-season (P < 0.05; Table 1).
Amino acid composition
A significant species × season interaction was observed for histidine, methionine, phenylalanine, arginine and proline among the essential AA (Table 2). The concentration of histidine was greater (P < 0.05) in A. mearnsii leaf-meal harvested in the hot-dry-season than A. dealbata × hot-dry-season leaf-meal but both were not different (P > 0.05) from both leaf-meal species harvested in the cool-wet-season (Table 2). Methionine concentration was lower (P < 0.05) in A. dealbata × hot-dry-season leaf-meal than in other leaf-meals (P < 0.05; Table 2). Phenylalanine concentration in Acacia leaf-meals was in the following order: A. mearnsii × cool-wet-season > A. mearnsii × hot-dry-season > A. dealbata × cool-wet-season > A. dealbata × hot-dry-season (P < 0.05; Table 2).
Table 2. Least square means of the concentration of amino acids in A. mearnsii and A. dealbata leaf-meals in the hot-dry and cool-wet seasons

s.e.m, standard error of means.
Arginine was lower (P < 0.05) in A. dealbata × hot-dry-season leaf-meal than in other interactions (Table 2). Concentrations of proline were greatest in the A. mearnsii × hot-dry-season leaf-meal followed by A. mearnsii × cool-wet-season leaf-meal and lowest in A. dealbata leaf-meals irrespective of the season (P < 0.05; Table 2). Species × season interaction had no effect (P > 0.05) on the remaining AA but were significantly influenced by both species and season except for serine which was influenced by species only (Table 2). The concentrations of isoleucine, leucine, serine, lysine, threonine, valine, glycine, tyrosine, alanine, asparagine, glutamine and serine were greater (P < 0.05) in A. mearnsii leaf-meals than in A. dealbata leaf-meals. The leaf-meals for the same AA except for serine had greater (P < 0.05) concentrations in the cool-wet-season than in the hot-dry-season (Table 2).
Mineral composition
Acacia species × season interaction influenced (P < 0.05) most of the analysed minerals, except for zinc (P > 0.05; Table 3). The content of calcium in leaf-meals was in the following order: A. dealbata × cool-wet-season > A. mearnsii × hot-dry-season > A. dealbata × hot-dry-season > A. mearnsii × cool-wet-season (P < 0.05; Table 3). Potassium and phosphorus leaf-meal contents were greatest in A. mearnsii × hot-dry-season followed by A. mearnsii × cool-wet-season, A. dealbata × cool-wet-season and A. dealbata × hot-dry-season in that order (P < 0.05; Table 3). Regardless of the season, A. mearnsii leaf-meals had the greatest contents of magnesium followed by A. dealbata leaf-meal harvested in the cool-wet and hot-dry-season in that order (P < 0.05; Table 3). Sodium concentration in Acacia leaf-meals was in the order of A. mearnsii × cool-wet-season > A. mearnsii × hot-dry-season > A. dealbata leaf-meals irrespective of the season (P < 0.05; Table 3).
Table 3. Least square means of the mineral contents in A. mearnsii and A. dealbata leaf-meals in the hot-dry and cool-wet seasons

s.e.m, standard error of means.
1 μg/kg.
Copper content was greatest in A. mearnsii leaf-meal in the cool-wet-season and lowest in A. dealbata leaf-meal in the hot-dry-season (P < 0.05; Table 3). The content of selenium was greater (P < 0.05) in A. dealbata leaf-meal in the cool-wet-season compared to the other interactions (Table 3). Iron was greatest in A. dealbata leaf-meal harvested in the cool-wet-season and lowest in A. mearnsii leaf-meal harvested in the hot-dry-season (P < 0.05; Table 3). The manganese content in the leaf-meals was in the order of A. mearnsii leaf-meal × cool-wet-season > A. dealbata × cool-wet-season > A. mearnsii × hot-dry-season > A. dealbata × hot-dry-season (P < 0.05; Table 3). The content molybdenum in leaf-meals followed the order of A. dealbata × cool-wet-season > A. dealbata × hot-dry-season > A. mearnsii × hot-dry-season > A. mearnsii × cool-wet-season (P < 0.05; Table 3). Aluminium content in leaf-meals was greatest in the A. dealbata × cool-wet-season leaf-meal, intermediate in the A. dealbata × hot-dry-season and lowest in A. mearnsii leaf-meals regardless of season (P < 0.05; Table 3). Regardless of the season, the zinc content of the leaf-meals was greater (P < 0.05) in A. mearnsii than A. dealbata. Cool-wet-season leaf-meal had greater (P < 0.05) zinc content than the hot-dry-season leaf-meal (Table 3).
Fatty acid composition
Acacia species × season interaction had a significant effect on all the analysed FA (Table 4). Palmitic (C16:0) and linoleic acid (C18:2n6) proportions in Acacia leaf-meals were greatest in A. mearnsii × hot-dry-season, intermediate for A. mearnsii × cool-wet-season and lowest in A. dealbata leaf-meals regardless of season (P < 0.05). The proportion of stearic acid (C18:0) was greatest in A. mearnsii leaf-meals irrespective of the season and lowest in A. dealbata leaf-meal in the cool-wet-season (P < 0.05). Acacia dealbata leaf-meal in the hot-dry-season had the greatest proportions of arachidic acid (C20:0) with A. mearnsii leaf-meal in the cool-wet-season having the lowest proportions (P < 0.05). The proportion of behenic acid (C22:0) was greatest in A. mearnsii leaf-meal harvested in the hot-dry-season and lowest in the leaf-meals harvested in the cool-wet-season regardless of species (P < 0.05). Total saturated fatty acids (SFA) were greatest in A. mearnsii leaf-meal harvested in the hot-dry-season followed by A. mearnsii × cool-wet-season and lowest in A. dealbata × cool-wet-season (P < 0.05). The proportions of oleic acid (C18:1n9) in leaf-meals followed the order of A. mearnsii × hot-dry-season > A. dealbata × hot-dry-season > A. dealbata × cool-wet-season > A. mearnsii × cool-wet-season (P < 0.05). Alpha-linolenic acid (C18:3n3) was greatest in A. dealbata leaf-meal in the cool-wet-season, and lowest in A. mearnsii leaf-meal in the hot-dry-season (P < 0.05). The proportion of γ-linolenic acid (C18:3n6) was greatest for leaf-meals harvested in the hot-dry-season irrespective of species and lowest in A. dealbata leaf-meal in the cool-wet-season (P < 0.05). Total polyunsaturated fatty acids (PUFA) were greatest in leaf-meals of A. dealbata × cool-wet-season and lowest in A. mearnsii × hot-dry-season (P < 0.05).
Table 4. Least square means of fatty acid contents in A. mearnsii and A. dealbata leaf-meals in the hot-dry and cool-wet seasons

s.e.m., standard error of means; SFAs, Saturated fatty acids (sum of C16:0, C18:0, C20:0 and C22:0); PUFAs, Polyunsaturated fatty acids (sum of C18:3n3, C18:2n6 and C18:3n6).
Phytochemical composition
Acacia species × season interaction had a significant effect only on quercetin-3-galactoside, p-Coumaroyltrifolin A and terpene lactone. Acacia mearnsii leaf-meal harvested in the hot-dry-season had a greater (P < 0.05) concentration of quercetin-3-galactoside relative to other leaf meals (Table 5). The concentration of p-Coumaroyltrifolin A and terpene lactone in leaf-meals were in the order of A. mearnsii × hot-dry-season > A. mearnsii × cool-wet-season > A. dealbata × hot-dry-season = A. dealbata × cool-wet-season (P < 0.05; Table 5). The concentration of (−)-epigallocatechin, p-Coumaroyltrifolin B, kaempferol 3-sophorotrioside and quercitrin were influenced by both species and season with, A. dealbata leaf-meals and cool-wet-season leaf-meals having greater (P < 0.05) concentrations than the other treatments (Table 5). For isorhamnetin, astilbin and 3-O-beta-glucopyranosyl-(1 > 6)-beta-glucopyranosyl-1-octen-3-ol, their concentrations were greater (P < 0.05) in A. mearnsii leaf-meals and cool-wet-season leaf-meals than the other treatments (P < 0.05; Table 5). Tataroside was influenced by species only with A. mearnsii leaf-meals having greater (P < 0.05) concentration than A. dealbata leaf-meals (Table 5).
Table 5. Least square means of the concentrations of phenols in A. mearnsii and A. dealbata leaf-meals in the hot-dry and cool-wet seasons
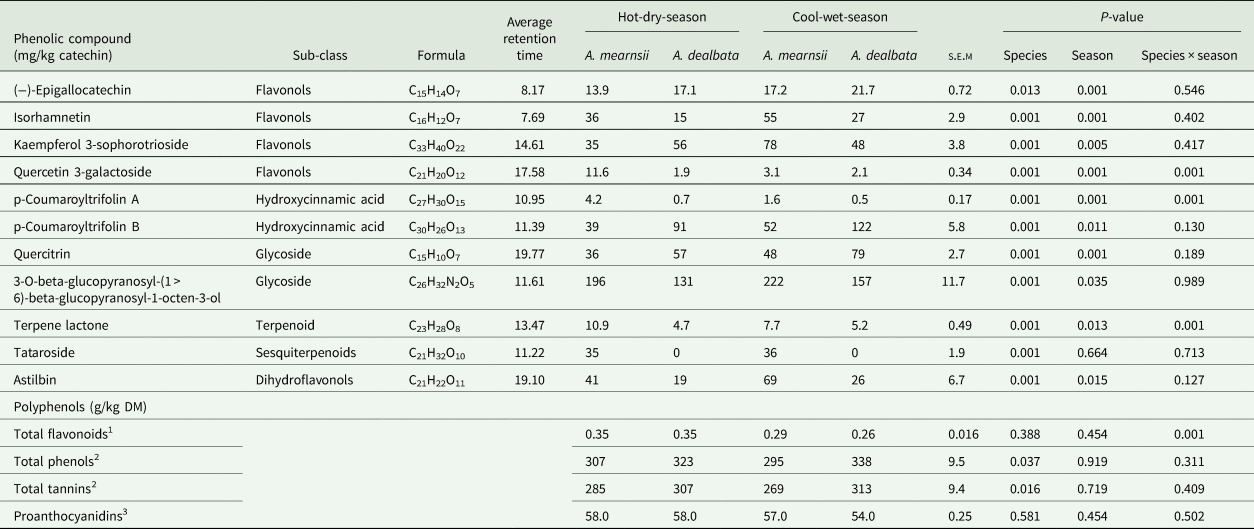
s.e.m., standard error of means.
1 Expressed as catechin equivalent.
2 Expressed as gallic acid equivalent.
3 Expressed as leucocynadin equivalent.
There was a significant interaction of Acacia species × season for total flavonoids concentrations, with A. mearnsii and A. dealbata leaf-meals harvested in the hot-dry-season having greater concentration than the other interactions (Table 5). The concentrations of total phenols and total tannins were only influenced by species with A. dealbata leaf-meals having greater (P < 0.05) concentrations than A. mearnsii leaf-meals (Table 5). No significant interaction of Acacia species × season was observed for proanthocyanidins.
Antioxidant activity
The effects of species, season and their interaction were not significant for DPPH (Table 6). Lipoxygenase and FRAP activity were not affected by Acacia species × season interaction (P > 0.05; Table 6), but individual effects of species and season were significant. Lipoxygenase activity was greater (P < 0.05) for A. mearnsii leaf-meals and hot-dry-season leaf-meals than in their counterparts (Table 6). Relative to their counterparts, A. dealbata leaf-meals and hot-dry-season leaf-meals had greater (P < 0.05) FRAP activity (Table 6). There was a significant interaction of species × season for APC index with the following order: A. dealbata × hot-dry-season > A. mearnsii × hot-dry-season > A. mearnsii × cool-wet-season > A. dealbata × cool-wet-season.
Table 6. Least square means of antioxidant activity of A. mearnsii and A. dealbata leaf-meals in the hot-dry and cool-wet seasons
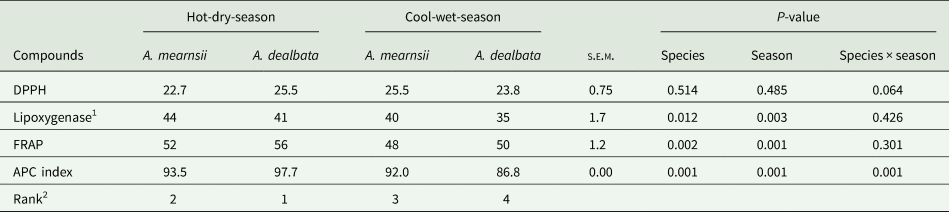
s.e.m, standard error of means; DPPH, 2-diphenyl-1-picrylhydrazyl expressed as % radical scavenging activity; FRAP, ferric reducing antioxidant power expressed as micromolar Trolox equivalent per gram DM; APC, antioxidant potency composite index.
1 Expressed as % inhibition of lipoxygenase activity.
2 Ranked according to the APC index, with 1 being higher antioxidant activity.
Discussion
The greater content of CP observed for A. mearnsii leaf-meal harvested in the hot-dry-season compared to other leaf-meals could be related to the interconnected effects of genetics and primary environmental stressors (i.e. high temperature, solar radiation and water deficit). Heat, solar radiation and water stress in the hot-dry-season might have induced specific genes in A. mearnsii to synthesise and elevate the accumulation of stress-specific proteins such as proline (Ul Haq et al., Reference Ul Haq, Khan, Ali, Khattak, Gai, Zhang, Wei and Gong2019; Karmous and Verma, Reference Karmous and Verma2020), which was high in the same leaf-meal in the current study. The elevated CP content observed for A. mearnsii leaf-meal in the hot-dry-season could also be partly related to the low fibre and tannin contents reported for the same leaf-meal, which may have led to low fibre-protein and tannin-protein complexes, hence increased free unbound protein molecules (Grabber, Reference Grabber2005; Naumann et al., Reference Naumann, Tedeschi, Zeller and Huntley2017). If fed as a sole diet, the range of CP content (165–249 g/kg DM) in the current study is sufficient to meet the CP (110–175 g/kg DM) requirements for productive ruminants (Freer et al., Reference Freer, Dove and Nolan2007).
The observation that A. mearnsii leaf-meal in the hot-dry-season had the greatest EE contents could be possibly related to genetics and seasonality in plant energy and fatty acid metabolism. The greater content of EE in A. mearnsii could be explained by a high content of chloroplasts due to its dark-olive green leaves compared to A. dealbata silvery-grey green leaves (Falcone et al., Reference Falcone, Ogas and Somerville2004; Aid, Reference Aid2019). Biosynthesis of FA occurs mostly in chloroplasts and in the plastids of non-photosynthetic tissues (Falcone et al., Reference Falcone, Ogas and Somerville2004; Aid, Reference Aid2019). The seasonal differences in EE can be explained by the fact that plants in the hot-dry-seasons optimise their energy metabolism by sequestering toxic FA degraded by membrane lipids that supply energy, while excess lipids are transformed into cutin and wax that prevent water loss and subsequently increases the lipid content of leaves (Falcone et al., Reference Falcone, Ogas and Somerville2004; Kim, Reference Kim2020). This is evident in the high unsaturated FA contents observed for A. mearnsii leaf-meal in hot-dry-season. The reported EE values (3.7–5.0 g/kg DM) in Acacia leaves are in the same range with those required for maintenance (i.e. 4.0–4.5 g/kg DM) in ruminants (Freer et al., Reference Freer, Dove and Nolan2007).
The observation that starch was greatest in A. dealbata × cool-wet-season leaf-meal compared to other interactions, could be possibly related to their differences in leaf morphology and seasonal response to light intensity and water availability which influence photosynthesis. Acacia dealbata has larger leaves (Weeds of Australia, 2016), hence large photon-harvesting surface and more stomata than A. mearnsii (Yan et al., Reference Yan, Zhong and Shangguan2017). Under low-light conditions prevalent in the cool-wet-season, plants develop a higher specific leaf area, which consequently increases photon-harvesting surface and stomata to optimise light capture and carbon gain for photosynthesis (Steinger et al., Reference Steinger, Roy and Stanton2003; Dong et al., Reference Dong, Fu, Liu and Liu2014; Liu et al., Reference Liu, Dawson, Prati, Haeuser, Feng and van Kleunen2016). Also, sufficient water in the cool-wet-season may increase stomata openings and subsequently increase carbon dioxide concentration in the mesophilic tissue that accelerates photosynthesis (Yan et al., Reference Yan, Zhong and Shangguan2017). The process of photosynthesis has been reported to increase the content of non-structural carbohydrates in leaves that lead to greater starch reserves in plants (Thompson et al., Reference Thompson, Gamage, Hirotsu, Martin and Seneweera2017). The present values for starch (75–178 g/kg DM) are lower than the readily fermentable carbohydrates values (230–280 g/kg) required for ruminant production (Freer et al., Reference Freer, Dove and Nolan2007).
The elevated plant fibre contents noted for A. mearnsii leaf-meal harvested in the cool-wet-season could be related to this species' superior ability to increase formation and deposition of phenolic polymers (i.e. lignin and suberin) in the cell wall to prevent itself from chilling injury and cell collapse under cold stress (Sharma et al., Reference Sharma, Shahzad, Rehman, Bhardwaj, Landi and Zheng2019). The greater content of aNDFom in A. mearnsii may also be ascribed to its faster growth rate compared to A. dealbata, which could have increased the accumulation of cell wall (Van Soest, Reference Van Soest1994). The aNDFom values for the Acacia leaf meals reported in the current study are above the acceptable range of 100–210 g/kg DM aNDFom required for optimal DMI (319 g/kg DM) and digestibility (520 g/kg DM) of browse legume forages in small ruminant diets (Hassan et al., Reference Hassan, Hafsa, Ibrahim and Mocuta2015). Elevated lignin (sa) content observed for A. dealbata leaf-meal in the hot-dry-season may be attributed to high temperatures and light intensity in the hot-dry-season that stimulates lignin synthesis in plants along with an increased concentration of phenylalanine ammonia lyase (PAL), a key enzyme in the biosynthesis of structural and defence compounds in plants (Nelson and Moser, Reference Nelson and Moser1994; Bhardwaj et al., Reference Bhardwaj, Handa, Sharma, Kaur, Kohli, Kumar and Kaur2014).
The findings that A. mearnsii leaf-meal in the hot-dry-season had greatest NDSF could be attributed to its low total tannin and fibre contents which could lessen the fibre-to-protein and tannin-to-protein ratios, resulting in less interlinking of soluble fibre with resistant protein and unbound tannins, thus increasing NDSF (Llobera and Cañellas, Reference Llobera and Cañellas2007). Furthermore, increased NDSF in hot-dry-season could be possibly due to plants ability to synthesise more soluble sugar-alcohols that substitute for water molecules in the hydration layer of proteins and membranes, thus allowing the functionality of enzymes at extremely low water concentrations (Figueroa et al., Reference Figueroa, Minen, Podestá and Iglesias2020). The higher coefficient ivNDFd observed at 24 and 48 h for A. mearnsii leaf-meal in the hot-dry-season compared to other leaf-meals could be related to its higher CP and lower aNDFom contents. A higher CP content supplies nitrogenous compounds that stimulate microbial growth on the fibrous carbohydrates, which leads to increased aNDFom digestibility (Souza et al., Reference Souza, Detmann, Paulino, Sampaio, Lazzarini and Filho2010).
The finding that A. mearnsii leaf-meal harvested in the hot-dry-season contained high proline content was expected since plants in the hot-season releases proline because of its osmoprotective properties and ability to detoxify reactive oxygen species (ROS), enabling plants to cope with environmental stresses and sustain plant growth (Kavi Kishor and Sreenivasulu, Reference Kavi Kishor and Sreenivasulu2014; Khan et al., Reference Khan, Ali, Zandi, Mehmood, Ullah, Ikram, Adnan Shahid and Babar2020). Generally, the cool-wet-season contained higher concentrations of most AA that may be attributed to the plants' ability to maintain basal levels of cellular metabolism at low temperatures by increasing dehydrogenase activity that leads to higher enzymatic activities, which accelerates protein synthesis (Guy, Reference Guy1990). The observation that A. mearnsii contained greater contents of AA may be an indication it is more thermally sensitive and code for more stress-tolerant genes as demonstrated by its capacity to produce more heat-shock proteins and protective proteins compared to A. dealbata (Ul Haq et al., Reference Ul Haq, Khan, Ali, Khattak, Gai, Zhang, Wei and Gong2019). Greater lysine content observed in A. mearnsii leaf-meals may be attributed to its higher proline content that also protects cellular structures, stability and integrity of proteins and increase enzyme activity (Karmous and Verma, Reference Karmous and Verma2020). Furthermore, the observation that lysine was lowest in the hot-dry-season may be related to its response to osmotic stress, which triggers its degradation to generate glutamic acid that act as a precursor of proline (Nakayama et al., Reference Nakayama, Kitada and Kinoshita1961; Arruda et al., Reference Arruda, Kemper, Papes and Leite2000). The lysine and methionine are usually limiting in ruminant production and contents reported in the current study do not meet the required levels for rumen microbes in growing ruminants for meat production at 0.32–0.9 and 0.26–2.9 g/day, respectively (Han et al., Reference Han, Ha, Lee, Ko and Lee1996; Klemesrud et al., Reference Klemesrud, Klopfenstein, Lewis, Methionine, Klopfenstein and Lewis2000; Lin et al., Reference Lin, Xiao, Zhao, Li, Xing, Li, Kong, Li, Zhang, Liu, Chen, Qin, Wu and Chen2016) and milk production at 18.7–22 and 59.3–68 g/kg DM, respectively (Rapetti and Bava, Reference Rapetti and Bava2005; Wang et al., Reference Wang, Liu, Wang, Yang, Liu, Wu, Yan and Ye2010).
The elevated contents of calcium and micro-minerals (i.e. selenium, iron, molybdenum and aluminium) observed for A. dealbata in the cool-wet-season leaf meal could be attributed to its observed higher contents of total phenols and phenolic compounds, particularly flavonols. In plants, phenolic compounds and their metabolites improve mineral absorption via chelation of metallic ions, enhancing active absorption sites and soil porosity, that accelerates the mobilisation of minerals (Halvorson et al., Reference Halvorson, Gonzalez, Hagerman and Smith2009; Sharma et al., Reference Sharma, Shahzad, Rehman, Bhardwaj, Landi and Zheng2019). Furthermore, it is reported that at low temperatures and high contents of soil moisture plants increase the production of minerals to suppress oxidative stress (Sharma et al., Reference Sharma, Shahzad, Rehman, Bhardwaj, Landi and Zheng2019). Also, A. dealbata silvery-grey green leaves compared to A. mearnsii dark-olive green leaves, may explain the higher content of immobile minerals such as iron that are observed to be high in leaves with less chlorophyll (Marschner, Reference Marschner1997).
The increased contents of potassium, magnesium and phosphorus reported for A. mearnsii leaf-meal in the hot-dry-season may be associated with high temperatures and evapotranspiration rates, which may have enhanced the uptake and translocation of mineral elements from the roots to the leaves (Marschner, Reference Marschner1997). Moreover, increased accumulation of the aforementioned minerals in plants during the hot-dry-season could be attributed to stomatal regulation by potassium ions that maintain water retention in plant tissues and corresponding higher rates of photosynthesis and mineral uptakes (Wang et al., Reference Wang, Zheng, Shen and Guo2013; Dias et al., Reference Dias, Guimarães, Neto, Silveira and Lacerda2017; Malhotra et al., Reference Malhotra, Sharma and Pandey2018). It may also be ascribed to faster growth rates of A. mearnsii compared to A. dealbata as mentioned earlier (Fangji et al., Reference Fangji, Chuanbi, Yongyuan, Huadong, Jiyuan and Yulin1994; Stelling, Reference Stelling1998). As the age and size of the plants increase, the relative importance of the evapotranspiration rate, particularly for the translocation of mineral elements increases (Marschner, Reference Marschner1997). Regardless of the season, A. mearnsii leaf-meals contained greater magnesium concentrations compared to A. dealbata, which could be related to the plant's magnesium remobilisation capacity by a breakdown of cell structures (e.g. chloroplasts) and enzyme proteins thereby transforming structurally bound mineral nutrients (e.g. magnesium in chlorophyll) into a soluble form in leaves (Marschner, Reference Marschner1997). It is noteworthy, that the calcium, phosphorus, magnesium and potassium contents of A. mearnsii and A. dealbata leaf-meals are within the recommended maintenance requirements for sheep and cattle (1.15–11.0, 0.9–3.8, 0.56–2.2 and 0.93–5.0 g/kg DM), respectively; (Freer et al., Reference Freer, Dove and Nolan2007; Pulina et al., Reference Pulina, Nudda, Battacone, Fancellu and Francesconi2008; Gomes et al., Reference Gomes, Oliveira-Pascoa, Teixeira, de Medeiros, de Resende, Yañez and Ferreira2011).
The high proportion of C18:3n3 acid and low proportions of C18:2n6, C18:1n9 and SFAs reported for A. dealbata leaf-meal in the cool-wet-season could be ascribed to low temperatures and light intensity that increases the synthesis of trienoic FA from dienoic, monoenoic and saturated FA by the respective FA desaturases (FADs) to attenuate oxidative stress by scavenging ROS (Cao et al., Reference Cao, Yang, Cai and Zheng2011; Das et al., Reference Das, Roychoudhury and Anjum2014; Dar et al., Reference Dar, Choudhury, Kancharla and Arumugam2017). At low temperature, the cell membrane changes from the solid-gel to liquid-crystalline phase, which is correlated to a higher ratio of unsaturated to SFAs, providing higher tolerance under cold climate (Routaboul et al., Reference Routaboul, Fischer and Browse2000; Cao et al., Reference Cao, Yang, Cai and Zheng2011). Contrary, proportions of C18:3n3 acid were low, while C18:2n6, C18:1n9 and SFAs were high for the A. mearnsii leaf-meal in the hot-dry-season, which might be associated with increased temperatures above 15°C that causes mutations or transformations in the FADs pathways (Falcone et al., Reference Falcone, Ogas and Somerville2004; Wiley et al., Reference Wiley, Figueiredo, Barroso, Pedro and Scheffer2008; Dar et al., Reference Dar, Choudhury, Kancharla and Arumugam2017). These changes modulate the level of desaturase activity at several steps within the lipid biosynthetic pathway as well as controlling inter-compartmental flux between the chloroplastic and cytosolic pathways, increasing FA production that is transformed into cutin and wax, enabling plants to tolerate and protect themselves against increased temperatures and light intensity (Falcone et al., Reference Falcone, Ogas and Somerville2004; Aid, Reference Aid2019; Kim, Reference Kim2020). Furthermore, water deficit and heat stress accelerate the kinetic energy and movement of molecules across membranes thereby loosening chemical bonds within molecules of biological membranes (Wahid et al., Reference Wahid, Gelani, Ashraf and Foolad2007; Salehi-Lisar and Bakhshayeshan-Agdam, Reference Salehi-Lisar and Bakhshayeshan-Agdam2016). This makes the lipid bilayer of biological membranes more fluid by either denaturation of proteins or an increase in unsaturated FA (Wahid et al., Reference Wahid, Gelani, Ashraf and Foolad2007; Salehi-Lisar and Bakhshayeshan-Agdam, Reference Salehi-Lisar and Bakhshayeshan-Agdam2016) as observed for A. mearnsii in the hot-dry-season leaf-meal. The increased SFAs in A. mearnsii leaf-meal in the hot-dry season may also be due to A. mearnsii's faster growth rate (Fangji et al., Reference Fangji, Chuanbi, Yongyuan, Huadong, Jiyuan and Yulin1994; Stelling, Reference Stelling1998) as discussed earlier. Fully developed leaves exposed to high temperatures produce more SFAs that increase the melting temperature of plasma membranes and thus reduces heat tolerance of the plants (Xalxo et al., Reference Xalxo, Yadu, Chandra, Chandrakar and Keshavkant2020). Such changes in FA composition, which are mediated mainly by the activity of FADs, allow the maintenance of the membrane fluidity at low and high environmental temperatures (Upchurch, Reference Upchurch2008; Jerónimo et al., Reference Jerónimo, Cachucho, Soldado, Guerreiro, Bessa and Alves2020). The greater proportions of C18:3n3 acid in A. dealbata leaf-meal in the cool-wet-season and C18:2n6 in A. mearnsii leaf-meal in the hot-dry-season provides an opportunity for its inclusion in ruminant diets. That may increase duodenal flow and tissue deposition of PUFA [i.e. C18:3n3, C18:2n6 and their biohydrogenation intermediates (i.e. rumenelic, rumenic and vaccenic acids)], which seem to have positive human health effects (Frutos et al., Reference Frutos, Hervás, Natalello, Luciano, Fondevila, Priolo and Toral2020; Vahmani et al., Reference Vahmani, Ponnampalam, Kraft, Mapiye, Bermingham, Watkins, Proctor and Dugan2020).
The high concentration of phenolic compounds for A. mearnsii leaf-meal in the hot-dry-season may be due to its chlorophyll content, since phenolics are biosynthesised in the chloroplast and mesophyll cells (Agati et al., Reference Agati, Brunetti, Di Ferdinando, Ferrini, Pollastri and Tattini2013). The phenolics concentration are further exacerbated by high temperature, water and/or nutrient deficiency increasing stomatal closure that depresses the photosynthesis rates and activate PAL activity (Agati et al., Reference Agati, Brunetti, Di Ferdinando, Ferrini, Pollastri and Tattini2013; Sharma et al., Reference Sharma, Shahzad, Rehman, Bhardwaj, Landi and Zheng2019; Šamec et al., Reference Šamec, Karalija, Šola, Vujčić Bok and Salopek-Sondi2021). The increased amounts of flavonoids, glucosides, terpenoid and hydroxycinnamic acid observed for A. mearnsii leaf-meal in the hot-dry-season is essential in the photoprotection of plants to prevent cell membrane damage, oxidative stress and cell death during hot-dry-seasons (Di Ferdinando et al., Reference Di Ferdinando, Brunetti, Agati and Tattini2014). They act as antioxidants by scavenging superoxide, hydrogen peroxide, hydroxide, singlet oxygen, or peroxy radicals (Agati et al., Reference Agati, Brunetti, Di Ferdinando, Ferrini, Pollastri and Tattini2013; Di Ferdinando et al., Reference Di Ferdinando, Brunetti, Agati and Tattini2014). To exemplify, p-Coumaroyltrifolin A, a hydroxycinnamate that was high in A. mearnsii leaf-meal harvested in the hot-dry-season is effective in absorbing ultraviolet-B radiation than flavonoids that have a greater ability to absorb visible light and UV-A wavelengths (Agati et al., Reference Agati, Brunetti, Di Ferdinando, Ferrini, Pollastri and Tattini2013; Sharma et al., Reference Sharma, Shahzad, Rehman, Bhardwaj, Landi and Zheng2019).
The reduced concentration of quercetin-3-galactoside in A. mearnsii leaf-meal harvested in the cool-wet-season probably illustrates restoration of A. mearnsii plant growth after light, heat and water stress events inflicted in the hot-dry-season. Increased quercetin production in plants is associated with water inadequacy and UV light stress (Olsen et al., Reference Olsen, Slimestad, Lea, Brede, Løvdal, Ruoff, Verheul and Lillo2009; Sharma et al., Reference Sharma, Shahzad, Rehman, Bhardwaj, Landi and Zheng2019). Generally, decreased temperature and increased UV-B radiation may cause plant cells to produce ROS that may damage lipids, deoxyribonucleic acid, structural proteins and other cellular structures (Cirak and Radusiene, Reference Cirak and Radusiene2019). As a result, higher UV-B radiation and lower temperature can stimulate the production of secondary metabolites with UV-B absorbing and ROS-scavenging qualities, such as phenolic acids, proanthocyanidins and flavonoids (Cirak and Radusiene, Reference Cirak and Radusiene2019). The greater concentrations of flavanols observed in the cool-wet-season than in hot-dry-season could be ascribed to differences in the metabolic response of the shikimate pathway and biosynthesis of phenolic compounds (Ancillotti et al., Reference Ancillotti, Bogani, Biricolti, Calistri, Checchini, Ciofi, Gonnelli and Del Bubba2015). In the cool-wet-season, lower temperatures induce PAL activity that increases the biogenesis of phenolic compounds (Rezende et al., Reference Rezende, Borges, Santos, Alves and Paula2015). This also corresponds with the high copper content reported for the cool-wet-season leaf-meals, which also activates the PAL pathway (Rezende et al., Reference Rezende, Borges, Santos, Alves and Paula2015).
The higher phenolic compounds reported for A. mearnsii might be attributed to its defence mechanism that probably code for more phenolic biosynthesis stress resistance genes (Ul Haq et al., Reference Ul Haq, Khan, Ali, Khattak, Gai, Zhang, Wei and Gong2019). The finding that A. dealbata leaf-meals respectively contained 8.5 and 10% more total phenol and tannins further confirms its increased phenotypic plasticity to multiple abiotic stresses compared to A. mearnsii (Sharma et al., Reference Sharma, Shahzad, Rehman, Bhardwaj, Landi and Zheng2019). The proanthocyanidin values observed in the current study are within the recommended threshold of 20–50 g/kg DM that positively affect DMI and nutrient digestibility (Patra and Saxena, Reference Patra and Saxena2011; Naumann et al., Reference Naumann, Tedeschi, Zeller and Huntley2017), suppress helminths (⩾20 g/kg DM; Huang et al.,. Reference Huang, Liu, Zhao, Hu and Wang2018) and alleviate bloat (5–30 g/kgDM; Li et al., Reference Li, Tanner and Larkin1996; Sottie et al., Reference Sottie, Acharya, McAllister, Thomas, Wang and Iwaasa2014) in ruminants.
The result that A. dealbata leaf-meal harvested in the hot-dry-season was more efficient in chelating iron and scavenging ROS according to APC index using lipoxygenase, and FRAP is mostly attributed to its polyphenolic contents, particularly flavonoids (Farag et al., Reference Farag, Abdel-Latif, Abd El Baky and Tawfeek2020). At high temperatures, the plant's antioxidant system is activated via the stimulation of the phenylpropanoid biosynthetic pathway that induces the synthesis of phenolic acids (Šamec et al., Reference Šamec, Karalija, Šola, Vujčić Bok and Salopek-Sondi2021). Increased flavonoids and phenolic acids in plants are associated with the deactivation of endogenous oxidative enzymes that prevent enzymatic oxidation (Jannat et al., Reference Jannat, Oveisi, Sadeghi, Hajimahmoodi, Behzad, Choopankari and Behfar2010), reduce ROS by quenching oxygen ions (Maffei et al., Reference Maffei, Mithöfer and Boland2007) and/or act as reducing agents, hydrogen donors, pro-oxidants and metal ion chelators (Pfukwa et al., Reference Pfukwa, Fawole, Manley, Gouws, Opara and Mapiye2019). The increased antioxidant activity reported for A. dealbata leaf-meal could be ascribed to its increased phenotypic plasticity (Sharma et al., Reference Sharma, Shahzad, Rehman, Bhardwaj, Landi and Zheng2019), as emphasised previously. Overall, the observed antioxidant activity in the current study is comparable to Xiong et al. (Reference Xiong, Grace, Esposito, Wang and Lila2016) who reported antioxidant activity of A. mearnsii leaves. The polyphenols (i.e. proanthocyanidin) values reported in the current study are within the range of 20–50 g/kg DM reported to extend oxidative shelf-life of meat (Luciano et al., Reference Luciano, Vasta, Monahan, López-Andrés, Biondi, Lanza and Priolo2011; Gesteira et al., Reference Gesteira, Oliveira, Silva, Ribeiro, Ribeiro, Pereira, Lanna, Pinto, Rocha, Vieira and Bezerra2018) and increase the antioxidant activity of milk (Di Trana et al., Reference Di Trana, Bonanno, Cecchini, Giorgio, Di Grigoli and Claps2015; Cabiddu et al., Reference Cabiddu, Delgadillo-Puga, Decandia and Molle2019; Delgadillo-Puga et al., Reference Delgadillo-Puga, Cuchillo-Hilario, León-Ortiz, Ramírez-Rodríguez, Cabiddu, Navarro-Ocaña, Morales-Romero, Medina-Campos and Pedraza-Chaverri2019). Research to evaluate worm burdens, fatty acid profile and oxidative shelf-life of meat and milk from ruminants fed diets containing these Acacia leaf-meals is indispensable.
Conclusions
Relative to other leaf-meals, A. mearnsii leaf-meal harvested in the hot-dry-season had the lowest aNDFom and ADFom content and the greatest contents of CP, EE, NDSF, 24 and 48 h ivNDFd, macro-minerals, individual polyphenols, C18:1n9 and C18:2n6. Acacia dealbata leaf-meal harvested in the cool-wet-season had the highest starch, NFC, microminerals and C18:3n3 whereas A. dealbata leaf-meal harvested in the hot-dry-season had the highest APC index. The greatest AA concentrations were contained in A. mearnsii leaf-meals irrespective of the season. Regardless of species, hot-dry-season leaf-meals had the highest total flavonoids concentration. Overall, A. mearnsii leaf-meal harvested in the hot-dry-season had the most desirable nutritional, polyphenolic and antioxidant profiles, making it a potentially suitable nutraceutical and preservative in ruminant production and product preservation, respectively. Further studies are warranted to determine the optimum inclusion levels of these Acacia leaf-meals in ruminant diets and their effects on nutrient digestibility and utilisation, animal production and product quality, which is necessary for the adoption and utilisation of these alternative ruminant feed resources.
Acknowledgements
The authors would like to thank the Management of Boschendal Wine Estate for allowing us to harvest Acacia trees from their Estate.
Financial support
This research was supported by the Regional Universities Forum for Capacity Building in Agriculture (RUFORUM) Graduate Training Assistantship programme as financed by Stellenbosch University and University of Namibia; the South African Research Chairs Initiative (SARChI) partly funded by the South African Department of Science and Technology (UID: 84633), as administered by the NRF of South Africa is acknowledged for extra funding; and the African-German Network of Excellence in Science (AGNES) for granting a Mobility Grant in 2019; the Grant is generously sponsored by German Federal Ministry of Education and Research and supported by the Alexander von Humboldt Foundation.
Conflict of interest
None.
Ethical standards
Ethical clearance for the study protocol was approved by the Animal Ethics Committee at Stellenbosch University (ACU-2019-11390).