The gastrointestinal (GI) tract plays a pivotal role in nutrition, serving as the interface between dietary intake and physiological functions. Its functions extend beyond digestion and absorption, as the GI tract also hosts a complex microbial community, known as the gut microbiota, which interacts with the host’s immune and nervous systems. This intricate interplay, often referred to as the microbiota-gut-brain axis, has been implicated in various aspects of health and disease(Reference Cryan, O’Riordan and Cowan1). Diet is a key modulator of the microbiota-gut-brain axis, and understanding its impact is crucial for developing targeted nutritional interventions(Reference Sandhu, Sherwin and Schellekens2). This review covers recent advancements in methodologies for assessing GI tract physiology, with a focus on gut motility, fermentation, and permeability, and their applications in nutritional research.
Reverse translational studies to provide mechanistic insight
A reverse translational study, often referred to as a ‘bedside-to-bench’ approach, presents a unique opportunity to leverage insights from both human and animal trials, enhancing our clinical and mechanistic understanding. These studies involve collecting samples such as faecal, blood, or colonic biopsy specimens during human trials, which are subsequently utilised in in vivo and/or ex vivo experimental protocols. While reverse translational designs can be time and resource-intensive, they enable the conduct of mechanistic investigations that would be challenging to achieve solely through human studies. Importantly, by utilising human samples, reverse translational studies offer results that are more directly applicable to real-life scenarios compared to studies relying solely on animal models.
Given the relevance of the microbiome in gastrointestinal physiology, the use of human faecal samples applied to reverse translational studies is one way in which human gastrointestinal tract and gut-brain physiology can be more carefully studied. Human-to-mouse faecal microbiota transplantation (FMT) has been used to understand the impact of the gut microbiome in a range of conditions including depression(Reference Zheng, Zeng and Zhou3), inflammatory bowel disease(Reference Jang, Kim and Joo4), and throughout pregnancy(Reference Koren, Goodrich and Cullender5). Following faecal collection from human participants, inoculation of the microbiome from the faecal samples to germ-free (without any resident microorganisms) mice allows for further understanding of the effects of the microbiome on behavioural studies, metabolome composition and immune markers. Recent guidelines for reporting on animal FMT studies have provided recommendations for protocol design and reporting which aims to improve the quality and reproducibility of studies using this technique(Reference Secombe, Al-Qadami and Subramaniam6).
Reverse translational FMT protocol can be applied in nutrition studies by assessing or modifying the diet of human subjects before faecal sample collection. Additionally, the diet of the animals receiving the FMT can be adjusted to investigate diet-microbiota interactions. For example, one study using a dietary intervention modifying fermentable carbohydrate (FODMAP) intake in patients with irritable bowel syndrome (IBS) showed that symptom response related to the dietary change was associated with alterations to the metabolome and decreased urinary histamine levels(Reference McIntosh, Reed and Schneider7). Subsequently, a reverse translational design was used whereby faecal samples from IBS patients collected during the interventions were then applied in vivo to germ-free mice fed custom-designed low and high-FODMAP diets to investigate the role of the gut microbiota on visceral hypersensitivity (i.e. pain)(Reference De Palma, Shimbori and Reed8). An interaction between diet and the microbiota was observed, where histamine production may have induced intestinal mast cell activation. This finding suggests that microbiota-targeted therapies aimed at histamine could alleviate pain in a subset of IBS patients(Reference De Palma, Shimbori and Reed8). These insights, derived from the reverse translational approach, represent novel findings not previously demonstrated in human trials focused on diet.
Another method involves using human faecal samples in ex vivo models. This approach was employed in a study where patients with IBS underwent a randomised cross-over trial of low and high-FODMAP diets(Reference Tuck, Omar and De Palma9). The supernatants from faecal samples collected in this trial were directly applied to mouse dorsal root ganglion (DRG) in patch-clamp recordings and to the lumen of mouse colonic tissue for afferent nerve recordings (Fig. 1). The aim was to investigate neurophysiological effects on pain signalling before and after dietary interventions. Reduced excitability of DRG neurones was observed with application of faecal supernatant from patients reporting symptom improvement with the low FODMAP diet intervention, a response not seen with the high-FODMAP diet or colonic biopsy supernatant application. This was further explored through afferent nerve recordings, where intraluminal perfusion of faecal supernatant from before the dietary intervention increased markers of visceral sensitivity, an effect that was lost with application of faecal supernatant from the low FODMAP intervention. Additionally, the use of histamine antagonists successfully blocked these effects, providing mechanistic insights into the impact of altering dietary FODMAP intake(Reference Tuck, Omar and De Palma9). These findings indicate that the interplay between diet and microbiota influences the production of luminal mediators that affect pain responses, highlighting insights not achievable through clinical study findings alone.
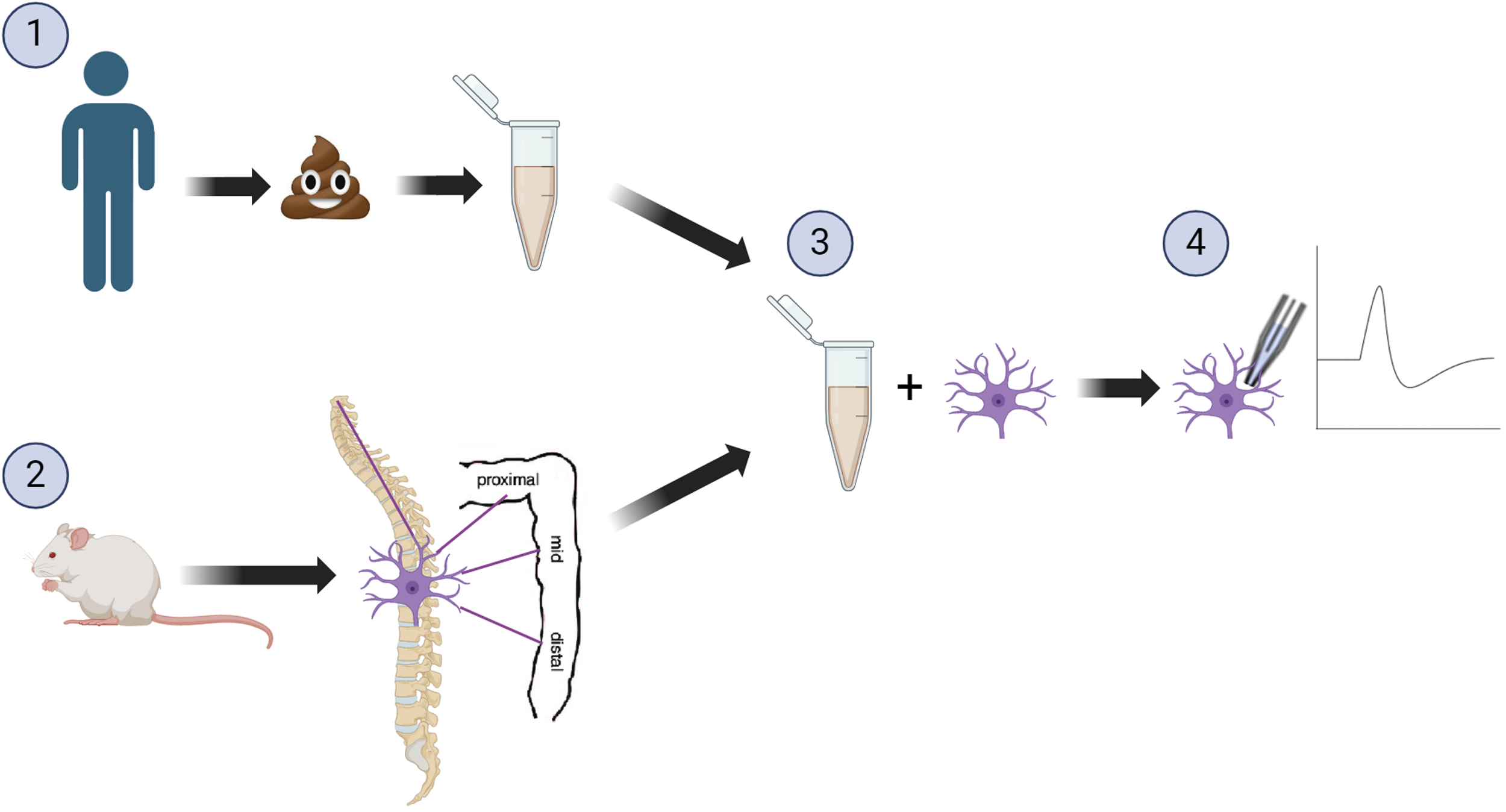
Figure 1. Example of a reverse translation study (1) Human participants receiving a dietary intervention, faecal samples collected and subsequently the supernatant was collected. (2) The dorsal root ganglion was collected from mice, and (3) incubated with the human faecal supernatant. (4) Patch-clamp recordings were taken, to assess the effects of the faecal supernatants following dietary intervention on neuronal excitability. Image created with biorender.com.
Although reverse translational designs utilising animal models offer distinct advantages, several critical design considerations must be addressed when employing such models in nutrition research. Firstly, there are notable physiological differences in the gastrointestinal tract among rodents, pigs, and humans that are essential for interpreting results accurately. For instance, when investigating changes in dietary intake of fermentable carbohydrates, it is crucial to consider the primary site of fermentation. In mice, fermentation predominantly occurs in the caecum, which is proportionally much larger compared to humans, in whom fermentation occurs in the colon(Reference Nguyen, Vieira-Silva and Liston10). Secondly, factors such as water source(Reference Barnett and Gibson11), the diet the animals consume(Reference Tuck, De Palma and Takami12), and even animal supplier(Reference Wolff, Jacobs and Haak13–Reference Tomkovich, Stough and Bishop15) have been shown to have a pronounced impact on the microbiome profile which has implications for reproducibility of results across laboratories. Large variations exist between mouse chows available and subsequently used across laboratories, including diets made from purified ingredients v. grain-based diets often formulated using ground wheat and corn. In a comparative study of these two distinct types of diet on caecal microbiota, notable differences in both α-diversity and β-diversity were observed(Reference Tuck, De Palma and Takami12). An alternative approach to using commercially available animal diets involves custom-designed animal diets that more closely mimic human consumption. This can be achieved for both rodents(Reference De Palma, Shimbori and Reed8,Reference Tuck, Caminero and Jiménez Vargas16) and pigs(Reference Kalantar-Zadeh, Yao and Berean17), with pig diets offering a closer representation to human dietary patterns through the use of whole-food ingredients. Interestingly, one study found that sourcing mice from different suppliers had an even greater effect on the microbiome than diet(Reference Rasmussen, de Vries and Kot14); thus to minimise supplier as a source of variation in the microbiome, it has been recommended that, where possible, animals be purchased in one shipment from one supplier(Reference Muralitharan, Snelson and Meric18). Thirdly, it is crucial that animal experiments consider dosages of test substrates that align with human consumption levels, rather than administering super-doses that exceed normal human dietary intake. This can be achieved through consideration of animal size and age alongside human dietary intake of the nutrient under investigation(Reference Lai, Chandrasekera and Barnard19), and should not be the maximal dose able to be administered as used in toxicology studies(Reference Committee20). Lastly, animal model limitations, such as coprophagy (the ingestion of faeces) which occurs in many commonly used animal models including mice, rats, guinea pigs, rabbits and pigs, must also be considered(Reference Nguyen, Vieira-Silva and Liston10). Coprophagy is a normal part of animal physiology and is important for the absorption of nutrients such as vitamin K and certain B vitamins, which are produced by the gut microbiota(Reference Savage21). Preventing coprophagic behaviour has been shown to alter animal physiology, particularly related to immunological tone(Reference Aviles-Rosa, Rakhshandeh and McGlone22–Reference Bo, Zhang and Kohl24). As a result of coprophagic activity, the gut microbiota of co-housed animals tends to become similar over time, resulting in a cage effect(Reference Muralitharan, Snelson and Meric18). One solution to mitigate this effect on experimental outcomes is to ensure that animals are only co-housed within the experimental group, and animals of different experimental groups are not co-housed together.
To fully leverage the potential of reverse translational study designs, enhanced multi-disciplinary integration of basic and clinical research is essential. Improved collaboration and understanding across these fields will lead to a more comprehensive grasp of the benefits offered by these combined techniques. Increasing the ability of clinicians, nutritionists, and dietitians to interpret fundamental scientific findings, and enabling discovery scientists to appreciate clinical relevance, including dietary manipulation, will result in higher-quality research outcomes.
Gastrointestinal tract motility
The complex process of GI motility, which encompasses the coordinated contractions and relaxations of the smooth muscles along the GI tract, is essential for the proper digestion and absorption of nutrients, mixing of luminal contents with digestive secretions, and the efficient propulsion and elimination of waste products. Abnormalities in GI motility, characterised by altered patterns of contractility, tone, or synchronisation, can lead to not only motility disorders but also various disorders of gut-brain interaction, such as IBS, functional dyspepsia (FD), and gastroparesis(Reference Black, Drossman and Talley25). These disorders not only significantly impair patients’ quality of life but also place a substantial burden on healthcare systems worldwide, with increased healthcare utilisation, productivity loss, and associated costs(Reference Sperber, Bangdiwala and Drossman26,Reference Aziz, Palsson and Törnblom27) . Therefore, accurate assessment of GI motility is crucial for understanding the pathophysiology of these disorders, developing targeted interventions, and monitoring treatment response in both clinical and research settings including diet-specific effects.
Recent advancements in technology have led to the development of novel tools for assessing GI motility in humans, providing a more comprehensive and detailed understanding of GI function compared to traditional methods(Reference Fox, Kahrilas and Roman28). Some of these tools include high-resolution manometry (HRM), wireless motility capsule,(Reference Thwaites, Yao and Halmos29) and MRI(Reference de Jonge, Smout and Nederveen30). Table 1 summarises the advantages and limitations including both established and emerging techniques. The choice of method depends on the specific research question and the targeted GI tract region. Detailed discussion of the latest tools for assessing gastric motility are provided below.
Table 1. Overview of gastrointestinal motility assessment: established and emerging tools
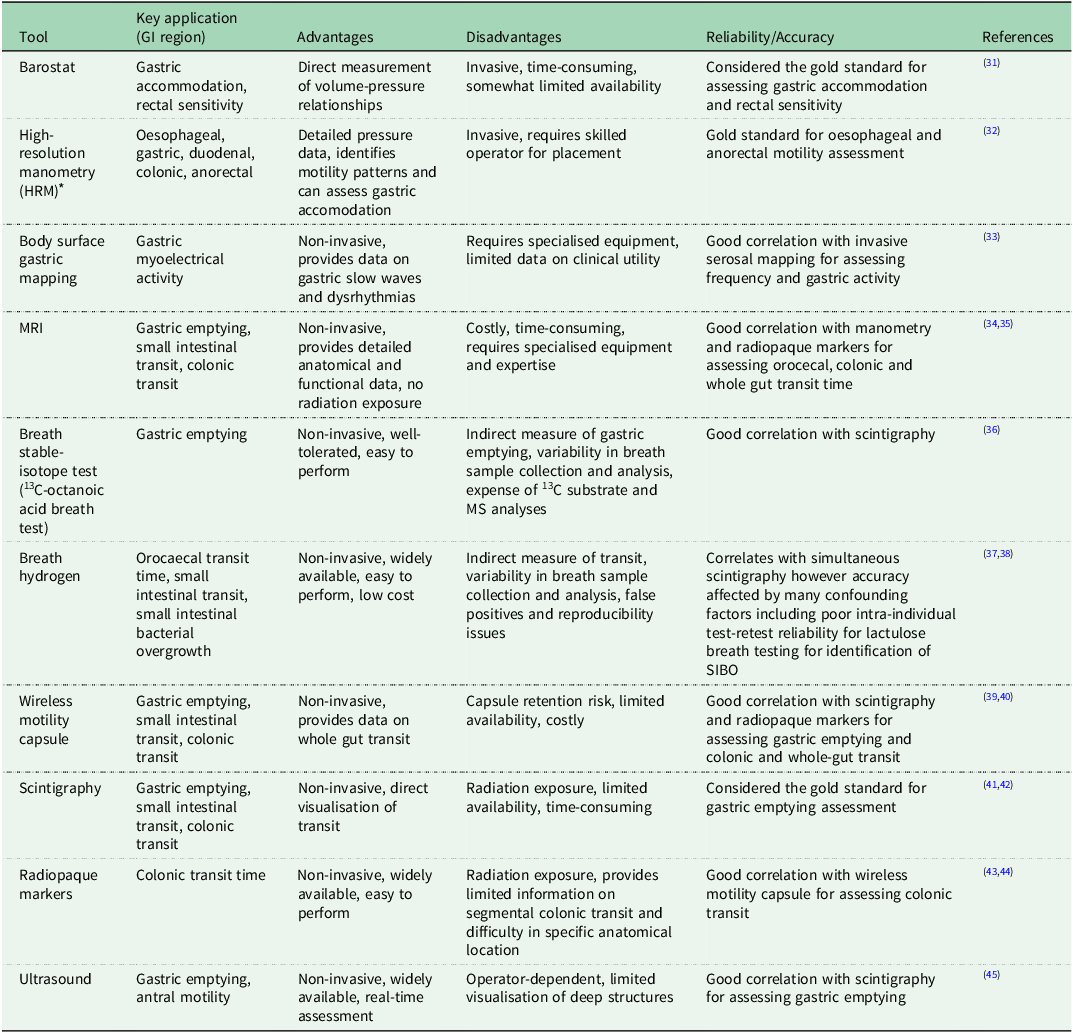
* Includes HRM with impedance, or with pH-impedance.
Gastric accomodation
Gastric function refers to the various activities and processes that occur within the stomach to aid in digestion and includes secretion of digestive enzymes for chemical digestion, gastric motility for mechanical digestion and gastric accommodation, which refers to the vagovagal-mediated reflex that occurs in the stomach during food intake. This reflex allows the stomach to relax and expand, providing a reservoir for the ingested meal before it is released into the small intestine in a controlled manner(Reference Febo-Rodriguez, Chumpitazi and Sher46,Reference Kindt and Tack47) . Impaired gastric accommodation is common in patients with FD and is associated with symptoms such as early satiety, postprandial fullness, and epigastric pain(Reference Tack, Piessevaux and Coulie48). The migrating motor complex (MMC) is another important aspect of GI motility, characterised by a cyclical pattern of electrical and contractile activity in the stomach and small intestine during fasting, consisting of three main phases: quiescent, irregular contractions, and regular, high-amplitude contractions(Reference Deloose, Janssen and Depoortere49). Control mechanisms for both gastric accommodation and MMC processes include hormonal and neural factors(Reference Deloose, Janssen and Depoortere49). Disruptions in the MMC have been implicated in various GI disorders, such as small intestinal bacterial overgrowth, IBS and FD, as well as non-GI disorders including diabetes and obesity(Reference Deloose, Janssen and Depoortere49).
Intragastric pressure measurement
High-resolution manometry (HRM) is a catheter-based technique that provides detailed pressure measurements along the length of each GI region(Reference Camilleri, Bharucha and di Lorenzo50). HRM provides a high spatial resolution and allows for the assessment of both the amplitude and propagation of peristaltic waves. This technique has revolutionised the understanding of GI motility disorders, particularly in the oesophagus, where it has become the gold standard for diagnosing and classifying motility disorders in clinical practice(Reference Tack, Pauwels and Roman51,Reference Yadlapati, Kahrilas and Fox52) . There are standardised HRM protocols to facilitate consistency and diagnostic reliability, detailing positions, manoeuvres, and importantly for oesophageal protocols, the bolus consistency(Reference Yadlapati, Kahrilas and Fox52).
In research settings, HRM has been a valuable tool for investigating the effects of various interventions, particularly liquid nutrient challenges, on gastric function. The measurement of intragastric pressure (IGP) during nutrient infusion using HRM has emerged as a promising alternative to the gastric barostat for assessing gastric accommodation and motility,(Reference Janssen, Verschueren and Ly53–Reference Carbone, Vanuytsel and Tack55) having shown good reliability when compared to barostat and scintigraphy(Reference Carbone, Tack and Hoffman56). This technique involves nasally inserting a thin HRM catheter and positioning it along the stomach’s length (from the fundus to the antrum and duodenum). The widespread use of HRM catheters in oesophageal manometry enhances its accessibility across various clinical and research settings. The protocol allows simultaneous measurement of IGP changes, nutrient volume tolerance and antral contractions during intragastric nutrient infusion, providing a comprehensive assessment of gastric function(Reference Janssen, Verschueren and Ly53,Reference Janssen, Verschueren and Tack54) .
For IGP measurements, participant preparation and data acquisition have been previously described, including in children and adults, healthy volunteers and different patient populations(Reference Janssen, Verschueren and Ly53–Reference Rotondo, Janssen and Mulè58). Participants lie semi-upright and can complete questionnaires (i.e. sensations of satiation and other subjective symptoms) or have blood samples taken throughout the experiment (e.g. concurrent hormonal measurements)(Reference Rotondo, Masuy and Verbeure59–Reference Meyer-Gerspach, Biesiekierski and Deloose62). Nutrient infusion is initiated after a stabilisation period (typically 10 min) at a low, constant rate (to avoid triggering secondary peristalsis)(Reference Janssen, Vanden Berghe and Verschueren63) until participants reach maximal satiation or report intolerable symptoms. Infusion should commence in late phase II of the MMC(Reference Deloose, Janssen and Depoortere49) to avoid interference with baseline measurements or strong phase 3 contractions. Participants can be easily blinded to the infusion contents, speed and volume to minimise psychological influences. Furthermore, the intragastric nutrient administration allows researchers to bypass any orosensory influences for food challenges. During infusion, the IGP exhibits a rapid decrease followed by a gradual recovery, indicating an initial gastric relaxation in response to nutrient infusion and a subsequent restoration of gastric tone(Reference Janssen, Verschueren and Tack54,Reference Papathanasopoulos, Rotondo and Janssen57,Reference Rotondo, Janssen and Mulè58) .
The advantages of IGP measurement using HRM is that it provides information on pressures in the lower oesophageal sphincter, proximal stomach, and distal stomach before, during, and after the meal. As well as using IGP to assess gastric accommodation, the HRM recording also allows for calculation of gastric and antroduodenal motility(Reference Meyer-Gerspach, Biesiekierski and Deloose62). Unlike the barostat, it does not interfere with the physiologically normal distribution of a meal within the stomach. However, like the barostat, HRM has limitations regarding invasiveness and laboratory setting measurements, which may not fully reflect real-world conditions. Participants must lie on a bed in a semi-upright position and the position of the HRM catheter (and any nasogastric tube) should be confirmed by fluoroscopy (real-time x-ray). In nutritional studies, HRM has been used to assess the effects of different liquid meal compositions or dietary components (e.g. peppermint oil,(Reference Papathanasopoulos, Rotondo and Janssen57) varying FODMAP concentrations,(Reference Masuy, Van Oudenhove and Tack64) nutritive v. non-nutritive sweeteners,(Reference Meyer-Gerspach, Biesiekierski and Deloose62) tastants(Reference Deloose, Janssen and Corsetti60,Reference Deloose, Vos and Corsetti61) ) on gastric accommodation, motility, and satiety. However, the technique’s sensitivity to detect subtle changes in gastric function induced by dietary interventions may be limited and measurements may be influenced by body position, respiration, and abdominal muscle activity, which should be considered when interpreting results(Reference Welch and Gray65).
Bioelectrical activity
Gastric electrical activity plays a central role in the organisation of gastric motility, particularly the slow wave, which is a propagating activation front that triggers the muscular contractions of peristalsis(Reference O’Grady, Du and Cheng66). Body surface gastric mapping (BSGM) is a non-invasive technique that has been developed to overcome the technical limitations of previous electrogastrography(Reference O’Grady, Varghese and Schamberg33) which lacks clinical utility(Reference Schamberg, Calder and Varghese67), and has been validated against invasive high-resolution serosal mapping(Reference O’Grady, Angeli and Du68). BSGM measures the cutaneous dispersion of gastric myoelectrical potentials, arising from extracellular ion current flows during depolarisation and repolarisation of gastric tissues(Reference Du, O’Grady and Cheng69). Recent advancements have enabled BSGM to provide valuable insights into the pathophysiology of altered gastric electrophysiology in the form of patterns of gastric dysrhythmias(Reference O’Grady, Angeli and Du68,Reference Angeli, Cheng and Du70) .
The first commercially available BSGM test, the Gastric Alimetry™ System (Alimetry, New Zealand), involves a 30-minute fasted recording, followed by a provocative test meal (482 kcal oatmeal bar and nutrient drink), and up to 4 h where patients sit in a reclined chair(Reference Carson, O’Grady and Du71–Reference Gharibans, Calder and Varghese73). Simultaneous digital symptom logging has recently enabled patient phenotyping alongside electrophysiology data(Reference O’Grady, Varghese and Schamberg33). Consensus working groups and other recent advancements in BSGM methods including the development of standardised test protocols, meals, output metrics, reference intervals, and disease classifications give promise for this technique to guide and inform clinical use(Reference O’Grady, Varghese and Schamberg33). However, as this technology is relatively new, some limitations include the somewhat inferred physiological basis of BSGM profiles and further data is needed to confirm relationships between gastric electrophysiology, motor activity, and symptoms(Reference O’Grady, Varghese and Schamberg33,Reference Carson, O’Grady and Du71) . In the context of future nutritional studies, ideally, BSGM should be able to be used to assess the postprandial response of gastric myoelectrical activity to different meal compositions and sizes, and importantly allow for varied meal completion. Currently, it is recommended that if less than 50 % of the test meal is consumed then BSGM data should be interpreted with caution(Reference Calder, Schamberg and Varghese72).
Imaging
MRI has emerged as a powerful tool for evaluating GI motility, offering a non-invasive, non-ionising method(Reference Marciani74). Recent advances in MRI technology, such as faster acquisition times, improved resolution, and dedicated post-processing software, have enabled the visualisation and quantification of global and segmental GI motility(Reference de Jonge, Smout and Nederveen30). MRI utilises the manipulation of hydrogen protons within the body using static and variable magnetic fields. Different pulse sequences generate contrast between tissue types, allowing for the assessment of various aspects of GI function, including gastric emptying, small bowel water content, and colonic volume(Reference Marciani74,Reference Khalaf, Hoad and Menys75) . Dynamic MRI, or cine MRI, involves acquiring a series of images to create movies of GI motion(Reference de Jonge, Smout and Nederveen30). Quantitative analysis of these images using post-processing techniques, such as the dual registration of abdominal motion implemented in the GIQuant software (Motilent Ltd, Ford, London), enables the assessment of global and local GI motility(Reference Menys, Taylor and Emmanuel76,Reference Menys, Hamy and Makanyanga77) .
Recent studies have demonstrated the utility of MRI in evaluating GI motility in healthy individuals and patients with various GI disorders(Reference de Jonge, Smout and Nederveen30). Gastric MRI has also been used to assess the effects of different dietary components, such as FODMAPs, on GI physiology and symptom generation in patients with IBS(Reference Major, Pritchard and Murray78) and uniquely interweaved with brain MRI to show how brain responses covary more extensively with GI symptom responses in IBS compared to gut responses(Reference Wu, Masuy and Biesiekierski79). Thus providing key data supporting the role of gut-brain axis dysregulation driving specific nutrient-induced symptom generation. However, MRI has some limitations, including supine positioning requirements, its high cost and time-consuming data processing(Reference Marciani74).
GI tract and fermentation
A key function of the colonic microbiota that contributes to host health and disease is the fermentation of carbohydrates and protein, of which diet is a major source. Approximately 12–40 g of poorly absorbed and indigestible carbohydrates and 12 g of undigested proteins arrive in the colon from a typical Western diet(Reference Cummings, Englyst and Wiggins80–Reference Yao, Muir and Gibson82), contributing to the substrate pool for fermentation. Fermentation of these carbohydrates generally produces gases (carbon dioxide (CO2), hydrogen (H2) and methane (CH4)) and short-chain fatty acids (SCFAs; acetate, propionate and butyrate) with resulting acidification of luminal contents and rapid expansion of the microbial biomass(Reference Cummings and Englyst83). However, as no poorly-absorbed or indigestible carbohydrates are created equal, the profile of metabolic end-products is highly dependent on the specific carbohydrate substrate(s) available to the microbes(Reference So, Gibson and Muir84). Conversely, fermentation of protein produces branched-chain fatty acids (BCFAs) and potentially toxic metabolites including ammonia, hydrogen sulphide (H2S) gas, phenols, indoles, and amines. These metabolic effects are also highly dependent on several factors including the type or amount of protein as well as the extent of carbohydrate fermentation(Reference Yao, Muir and Gibson82). Hence, to understand the fermentative profiles of the colonic microbiota in response to dietary interventions/manipulation in nutritional studies, there are a growing number of technological advancements that enable indirect or direct profiling of one or several of these metabolites simultaneously.
In vitro fermentation systems
In vitro fermentation systems are an accessible, low cost and rapid assay for simulating colonic fermentation in the laboratory, replacing in vivo. techniques. These systems enable gas release, SCFAs and pH to be measured simultaneously, allowing an overview of the metabolic output of the gut microbiota to be profiled in response to diet. Examples of their utility include screening the fermentation characteristics of a large number of dietary substrates such as novel fibres(Reference So, Yao and Gill85) or food additives(Reference Gerasimidis, Bryden and Chen86) to predict their physiological response in vivo., examining interactions between dietary components (e.g. protein v. fibre or slowly fermentable psyllium v. readily fermentable inulin) on fermentation and investigating potential functional alterations of the gut microbiota in disease states. There are several in vitro. fermentation models that have been utilised with varying complexity ranging from in vitro. simulators such as the Simulator of the Human Intestinal Microbial Ecosystem (SHIME) model(Reference Molly, Woestyne and Smet87), a 3-stage continuous system that mimics the differences in microbial composition and activity across colonic regions to simple batch fermentation models that are static. The strengths and limitations of each method have been reviewed comprehensively elsewhere(Reference Ou, Yao and Rotbart88,Reference Kalantar-Zadeh, Berean and Burgell89) . In the design of in vitro. fermentation experiments, several aspects including faecal inoculum, substrates and duration of experimentation are highly influential in generating reliable results.
Faecal inocula
In the preparation of the faecal inocula, faecal samples from several participants are often pooled to obtain a representative diversity of the gut microbes in the colon. There is a growing body of evidence to suggest that the gut microbiota composition and metabolic responses can be heterogeneous in healthy individuals(Reference Cantu-Jungles, Bulut and Chambry90) as well as in disease states such as inflammatory bowel disease(Reference Armstrong, Bording-Jorgensen and Santer91). However, Aguirre et al. (Reference Aguirre, Ramiro-Garcia and Koenen92) demonstrated a similar cumulative production of SCFA between the pooled v. individual faecal samples, indicating a significant functional overlap but a higher biodiversity in the pooled samples. Interestingly, a large degree of inter-individual variability was seen in the production of BCFA, which was not present in the pooled samples. Therefore, the choice of pooling faecal samples or using individual inoculum may depend on the research question. Whether the faecal samples are collected fresh or frozen, is an important consideration, as the freeze-thaw process prior to the preparation of the faecal inocula has been shown to result in significant loss of specific gram-negative bacteria as well as other microbial communities(Reference Aguirre, Eck and Koenen93) and favour the growth of bacteria within Enterobacteriaceae family(Reference Poppe, Vieira-Silva and Raes94). Whilst freshly passed faecal samples are preferable to achieve optimal results, this may be impractical, and where freezing samples is unavoidable, cryopreservation with glycerol may attenuate unwanted effects on the microbial consortium(Reference de Carvalho, Oliveira and Dib Saleh95).
Substrates
The source of the dietary substrate can have significant implications for the fermentation result. Divergent fermentation characteristics of the same fibre substrate (arabinoxylans) but with slightly different chemical structures (i.e. from different classes of wheat) have been reported(Reference Tuncil, Thakkar and Arioglu-Tuncil96), a finding that was also observed by So et al. (Reference So, Yao and Gill85) with two different sources of xylo-oligosaccharides (almond v. corn) and different products of Hi Maize resistant starch type 2. This could explain some of the inconsistencies seen in the literature on fermentation profiles of a fibre substrate but highlights the importance of detailing either fibre structure or source of substrate.
Experimental duration
The duration of the fermentation experiments is an additional aspect of consideration. The majority of in vitro. experiments measure changes in fermentation kinetics over 24–48 h(Reference Ou, Yao and Rotbart97). A rapid 4-hour faecal gas profiler has been proposed, as one study showed a 3-unit drop in pH following incubations with fibre substrates at 4 h, which can have a dramatic impact on the metabolic activities of the gut microbiota. This was demonstrated in a 48-hour in vitro. study where the ability of microbes to ferment protein and generate phenols, a by-product of protein fermentation, decreased by 33 % at pH 5·5 v. pH 6·8(Reference Smith and MacFarlane98). Additionally, there may be differences in metabolic output in assays that are run for 24 h v. 4 h. For example, psyllium is ranked as a moderately fermentable fibre at 24 h based on its gas production profile(Reference Timm, Stewart and Hospattankar99), but minimally fermentable at 4 h(Reference So, Yao and Gill85). Therefore, batch fermentative experiments that are conducted over a prolonged duration, may have limited physiological relevance to what occurs in vivo. Hence, to overcome this limitation, a rapid 4-hour faecal gas profiler using in vitro. batch fermentation techniques was developed that enabled automated sampling of headspace gas release through an array of gas sensors that measure H2, CH4, H2S and CO2 in real-time, with data communicated wirelessly to a computer for analysis(Reference Rotbart, Yao and Ha100). This system has been demonstrated to be reliable and has good repeatability(Reference Yao, Rotbart and Ou101).
In vivo measurements: indirect profiling via breath testing
Breath measurements of H2, CO2 and CH4 are highly attractive due to their ease of testing and patient acceptability. H2 and CH4 are produced specifically in response to microbial fermentation of carbohydrates, whereas CO2 can diffuse into the gastrointestinal lumen from various chemical reactions in the GI tract (e.g. interactions between bicarbonate and stomach acid) but also from microbial fermentation. The premise of this test lies in the production of these gases in the colon that are then absorbed and travel to the lungs to be expelled in breath. Breath tests are usually performed to assess the malabsorption of carbohydrates such as in the assessment of lactose malabsorption, or the degree of fermentation in response to diets containing high or low fermentable carbohydrates such as FODMAPs. There are limitations with the use of breath hydrogen or methane assessment for carbohydrate malabsorption which may influence the interpretation of results. First, Yao et al. observed significant variability (8·5–345 %) in H2-producing capacity with breath testing performed on two separate occasions, at least 2 weeks apart following ingestion of 15 g lactulose, a non-digestible carbohydrate(Reference Yao, Tuck and Barrett102). Such large variations could potentially be explained by the hastening of transit that occurs with ingestion of lactulose as has also been demonstrated previously, but could also highlight differences in hydrogen utilisation in other hydrogen disposal pathways. Second, breath H2 and CH4 are measured in parts per million and are poorly sensitive to low levels of gas production. For example, in a study using varying amounts of inulin, breath H2 concentrations were not detectable when amounts of inulin less than 10 g were consumed(Reference Berean, Ha and Ou103). Despite this, measurements of breath H2 and CH4 remain valuable in research settings to semi-quantitatively assess fermentative responses to a novel fibre or interactions between fibre combinations and fermentation (e.g. psyllium attenuating gas production of inulin(Reference Gunn, Abbas and Harris104)), or as indicators of compliance in dietary interventions that manipulate carbohydrate/fibre intake.
In vivo measurements: direct profiling
Differences in metabolic activities of the colonic microbiota across the different colonic regions were first described by Macfarlane et al. (Reference Macfarlane, Gibson and Cummings105) following direct measurements of luminal contents of cadavers. Concentrations of SCFA were highest in the proximal colon(Reference Macfarlane, Gibson and Cummings105,Reference Cummings, Pomare and Branch106) , indicating that carbohydrate fermentation is most active in this region. As fermentable carbohydrate supply decreases along the length of the colon, by-products of protein fermentation, such as BCFA and phenols, increase in concentration(Reference Macfarlane, Gibson and Cummings105), suggesting that protein fermentation is more active in the distal colon. Additionally, different fibre types, depending on the amount available and its fermentability can shape the metabolic profile in specific colonic regions. For example, oat bran, a fermentable fibre that is fermented at a moderate rate, raised total SCFA only in the caecum and not in the distal colon, suggesting that its fermentation is exhausted by the end of the proximal region(Reference McIntyre, Young and Taranto107). In contrast, wheat bran, being fermented at a slower rate, increases fermentation along the length of the colon(Reference McIntyre, Young and Taranto107). Similarly, in a landmark study with pigs, supplementation with resistant starch increased butyrate production in the proximal colon, but the addition of wheat bran to resistant starch marked increased faecal SCFA concentrations, suggesting that fermentation was ‘carried’ to the distal colonic regions(Reference Govers, Gannon and Dunshea108). These concepts are of significant interest to nutrition studies where modulation of fermentation sites, as well as the delivery of metabolites to a specific colonic region, may have implications for optimising gut health.
Since the pioneering work of Macfarlane et al. (Reference Macfarlane, Gibson and Cummings105), technological advancements have evolved significantly with the advent of radiotelemetry devices including a wireless pH-motility capsule and more recently, intestinal gas using an Atmo gas-sensing capsule that can be performed in an ambulatory setting(Reference Thwaites, Yao and Halmos29). These telemetric capsules sample luminal contents via a semi-permeable membrane, enabling measurement of real-time changes in pH or gases directly at the site of fermentation(Reference Thwaites, Yao and Halmos29). Transit is also measured concurrently to enable localisation of the gastrointestinal region (e.g. stomach, small v. large intestine). These capsules therefore address current limitations of existing methods such as faecal SCFA measurements and breath H2 that provide an overview of fermentation, or in the case of faecal SCFA, only reflect fermentative events in the distal colon.
The site and extent of fermentation at each colonic region was first characterised using the wireless pH-motility capsule in 33 healthy controls as well as in individuals with IBS(Reference Ringel-Kulka, Choi and Temas109). Localisation of different colonic regions was performed by dividing the colon into quartiles based on transit time data. Using this approach, colonic pH levels were observed to be the lowest in the first quartile, confirming that fermentation was occurring maximally in the proximal region and pH increasing along the length of the colon. As a result, greater microbial fermentation was identified across all regions of the colon in patients with IBS compared to healthy controls. Subsequently, two studies(Reference So, Yao and Gill110,Reference Yao, Burgell and Taylor111) have utilised dietary fibre strategies to target changes in regional fermentation. First, direct measurements of pH using the wireless pH-motility device showed that feeding healthy controls high (13 g/d) amounts of fructans and resistant starch over a 24-hour period increased acidification of luminal contents along the length of the colon as well as in the distal colon compared to a low fibre diet (<2g)(Reference Yao, Burgell and Taylor111). Second, tandem measurements of a gas-sensing capsule and the wireless pH-motility device saw differences in patterns of fermentation in response to diets supplemented with no fibre, a minimally fermentable or a combination of fibres(Reference So, Yao and Gill110). For example, the wireless pH-transit capsule saw an increase in pH along the colon regardless of the fibre(s). In contrast, the gas-sensing capsule captured a significant increase in hydrogen in the last quartile of the colon, suggesting that fermentation has shifted distally compared to the no or poorly fermentable fibre where fermentation was mainly focused in the proximal colon(Reference So, Yao and Gill110). Both these studies expanded our understanding of how diet can be manipulated to exert effects in specific colonic regions and the use of such telemetric technology to confirm the desired effects.
There are certain limitations with the selection of participants that may hinder the application of these ingestible capsules including the presence of strictures in patients with inflammatory bowel disease, previous abdominal surgery or those with difficulties swallowing large tablets(Reference Thwaites, Yao and Halmos29). Furthermore, the ingestion of these capsules follows a standardised protocol validated for transit measurements. This involves participants being fasted overnight before coming into the research centre to consume a low energy, low-fat test meal containing 260 kJ with 2–3 % fat content. This is followed by a 6 h fast before the participant resumes usual activities. They will be instructed to wear a data receiver for the length of the capsule study until the capsule is passed(Reference Wang, Mohammed and Farmer112). Deviations in standardised protocol, either by replacing the standardised bar with a study meal that has a higher energy or fibre content(Reference Wallace, Eady and Drummond113), or by shortening the fasting period(Reference So, Yao and Gill110) may affect whole-gut transit assessments, specifically delayed gastric emptying but does not affect capabilities to capture fermentative changes. Finally, the costs of purchasing equipment, transmission issues, and participant compliance are all factors that may influence the sample size or quality of data collected. Recently, the use of blue food dye has been utilised to assess whole-gut transit time; this methodology has several benefits in that it is low cost, low participant burden and does not require specialised equipment(Reference Asnicar, Leeming and Dimidi114). The emergence of direct profiling presents an exciting avenue for diet and gastroenterology research, providing indicators for which dietary strategies may target region-specific fermentative capacity.
Intestinal permeability
The human GI tract is estimated to have a surface area of around 32m2(Reference Helander and Fändriks115). Across this surface, the GI tract must provide sufficient absorption of nutrients, electrolytes, and water from the lumen whilst also acting as a barrier against the entry of harmful substances, such as pathogens and toxins(Reference Buckley and Turner116). To achieve this barrier protection function, the GI tract is lined with a layer of epithelial cells, which are held together closely by tight junctions, adherens junctions, and desmosomes. In addition, this layer of cells contains goblet cells, which produce a thick layer of mucus, creating a physical barrier on top of the epithelial cell layer to prevent the gut microbiota from reaching the epithelial layer(Reference Cornick, Tawiah and Chadee117). This mucus layer consists of a thick inner layer of mucus, located proximally to the epithelial layer, and a thinner outer layer of mucus. This thinner outer layer of mucus serves as a niche where gut bacteria that are mucus degraders, such as Akkermansia muciniphila, can reside and degrade mucin glycans(Reference Luis and Hansson118). Together this layer of epithelial cells and mucus layer act as a physical barrier, preventing the translocation of bacteria and bacterial components that inhabit the lumen.
Disruption of the intestinal barrier can permit the passage of microbial components, such as lipopolysaccharide, into the lamina propria and subsequently into the systemic circulation. Disruption of the intestinal barrier leads to the activation of immune response pathways in the intestinal tissue itself(Reference Desai, Seekatz and Koropatkin119) and systemically(Reference Di Vincenzo, Del Gaudio and Petito120). Increased gut permeability is observed in many gut-related conditions such as ulcerative colitis and Crohn’s disease(Reference Nalle and Turner121). Furthermore, disruption of the GI barrier has been implicated in the chronic low-grade inflammatory processes that are observed with many non-GI conditions, such as diabetes(Reference Snelson, de Pasquale and Ekinci122), hypertension(Reference Snelson, Vanuytsel and Marques123) and kidney disease(Reference Meijers, Farré and Dejongh124). There is increasing evidence for the role of GI permeability in the pathogenesis of many health conditions(Reference Snelson, de Pasquale and Ekinci122,Reference Bischoff, Barbara and Buurman125) and is also affected by nutritional factors such as parental nutrition(Reference Purandare, Offenbartl and Weström126), degree of food processing(Reference Snelson, Tan and Clarke127), dietary emulsifiers(Reference Chassaing, Koren and Goodrich128), fibre(Reference Cani, Possemiers and Van de Wiele129), fat(Reference Rohr, Narasimhulu and Rudeski-Rohr130) and protein(Reference Snelson, Clarke and Nguyen131) content.
Transit across the gut barrier
Molecules in the lumen can pass across the GI epithelium through either paracellular or transcellular pathways, depending on the size, charge, and hydrophobicity of the molecule in question. Transcellular transport may be passive, where small compounds diffuse across the cell membrane; active, utilising cell surface receptors (e.g. PepT1 for the absorption of amino acids); or via endocytosis, where compounds such as larger peptides and proteins are enclosed in vesicles and absorbed. Importantly, this transcellular endocytosis appears to be one key mechanism by which bacterial lipopolysaccharide, and even whole bacteria themselves, can cross the intestinal epithelium(Reference Horowitz, Chanez-Paredes and Haest132,Reference O’Donoghue and Krachler133) . The epithelial cells of the GI tract are kept in close contact by tight junctions, adherens proteins and desmosomes, with the tight junctions (e.g. occludin, zonula occludens-1, and claudin-2) playing an important role in regulating the ability for molecules to pass between epithelial cells, known as paracellular permeability(Reference Campbell, Maiers and DeMali134). This paracellular pathway can be divided into the pore pathway, which permits passage of molecules <8 Å in size and the leak pathway which allows molecules up to ∼100 Å in size(Reference Zuo, Kuo and Turner135). An in-depth description of these two pathways, has been recently published by Horowitz et al. (Reference Horowitz, Chanez-Paredes and Haest132). When discussing intestinal permeability, it is the paracellular leak pathway and transcellular endocytosis that are most relevant, with the majority of research to date focused on paracellular mechanisms(Reference Vanuytsel, Tack and Farre136).
Measurement of intestinal permeability
Broadly, techniques for the assessment of intestinal permeability can be classified as either being a direct or indirect measurement. Direct, or functional, tests work on the principle of measuring the transit or flux of a molecule or group of molecules from one side of the epithelial barrier to the other, typically the luminal side to the basolateral side. A summary of the main methods utilised for directly measuring intestinal permeability, along with their respective advantages and disadvantages, is provided in Table 2. Indirect markers include the use of biomarkers in commonly collected samples such as plasma, that are purported to relate to intestinal permeability.
Table 2. Overview of methods utilised for directly measuring intestinal permeability
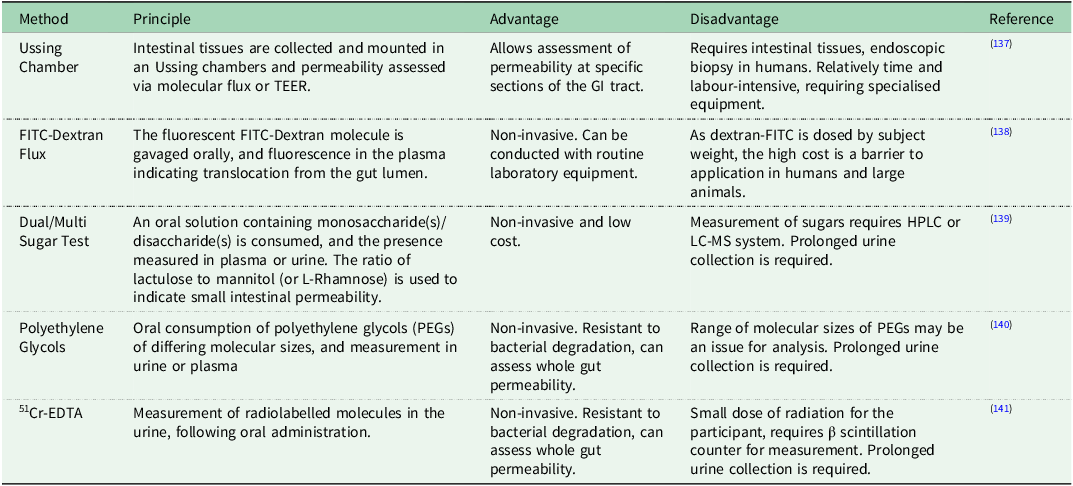
Legend: EDTA: Ethylenediaminetetraacetic Acid, FITC: Fluorescein Isothiocyanate, HPLC: High-Performance Liquid Chromatography, LC-MS: Liquid Chromatography-Mass Spectrometry, PEGs: Polyethylene Glycols, TEER: Transepithelial Electrical Resistance.
Direct measurements of intestinal permeability
In vivo assessment of intestinal permeability is commonly conducted via the oral consumption of probes and the measurement of those probes in the plasma and/or urine. Commonly utilised probes include polyethylene glycols (PEGs), Fluorescein Isothiocyanate (FITC)-Dextran, Chromium-51-labelled ethylenediaminetetraacetic acid (51CrEDTA), mannitol, lactulose, L-rhamnose, sucralose and sucrose(Reference Vanuytsel, Tack and Farre136). Tagesson et al. (Reference Tagesson, Sjödahl and Thorén142) first described the use of the fluorescent compound FITC-Dextran to measure intestinal permeability in 1978. This assay is widely used in small animal studies, including in mice(Reference Snelson, Tan and Higgins143), rats(Reference Purandare, Offenbartl and Weström126) and poultry(Reference Liu, Teng and Kim144). Dosing is typically done according to body weight or lean body mass(Reference Voetmann, Rolin and Kirk138), and while this technique could be applied to larger animals including humans, it typically is not, likely due to the increased dose, and associated cost, that would be required. PEGs can span a range of sizes, with molecular weights between 400 and 4000 typically utilised in studies of intestinal permeability, which are then measured in urine or plasma by high-pressure liquid chromatography(Reference Camilleri140). 51CrEDTA has been used in rodents(Reference Ten Bruggencate, Bovee-Oudenhoven and Lettink-Wissink145) and people(Reference Ten Bruggencate, Bovee-Oudenhoven and Lettink-Wissink146–Reference Arslan, Atasever and Cindoruk148) for the assessment of intestinal permeability, with the major disadvantage to this technique being the fact that participants do get exposed to a small amount of radiation(Reference Horton, Wright and Smith149), although a novel protocol using non-radioactive 52Cr-EDTA has recently been proposed(Reference von Martels, Bourgonje and Harmsen141). 51CrEDTA and PEGs are resistant to microbial fermentation and thus can measure whole-gut permeability(Reference Bjarnason, Macpherson and Hollander150).
By far the most common method for assessing intestinal permeability in vivo in people is the dual sugar test. The dual sugar test utilises a monosaccharide (typically mannitol or L-rhamnose) and a disaccharide (typically lactulose) that is not metabolised in the small intestine. The smaller monosaccharide is capable of passing through the paracellular pore pathway, and its presence in the plasma or urine is related to the small intestinal surface area(Reference Zuo, Kuo and Turner135). The disaccharide cannot pass through the pore pathway, instead, it travels via the leak pathway, and thus its presence in blood or urine signifies an increase in permeability of the paracellular leak pathway, with this typically expressed as a ratio between the disaccharide and monosaccharide, i.e. the lactulose mannitol ratio(Reference Vanuytsel, Tack and Farre136). Mannitol is a dietary contaminant, with 13C mannitol and L-rhamnose being increasingly utilised to avoid the issues associated with the presence of (12C) mannitol in the diet(Reference Khoshbin, Khanna and Maselli139,Reference Grover, Camilleri and Hines151) . The presence of these sugars in the urine up to two hours following oral consumption is considered to represent small intestinal permeability(Reference Camilleri140,Reference Seikrit, Schimpf and Wied152) , however, some studies do utilise urine collection periods of up to 5–6 h(Reference Wilms, Jonkers and Savelkoul153,Reference Seethaler, Basrai and Neyrinck154) . Whilst the majority of studies do measure the presence of these sugars in the urine, it has been suggested that serial plasma measurements provide greater sensitivity, particularly to transient changes in intestinal permeability that may be missed with bulk urine collection(Reference Houghton, Snipe and Williamson155). The probes utilised in the dual sugar test (lactulose, mannitol and L-rhamnose) are fermented by the colonic microbiota and while useful for assessing small intestinal permeability, are unreliable for colonic permeability.
It is increasingly recognised that there are vast differences between the small intestine and colon in terms of bacterial content, physiological functions and pH(Reference McCallum and Tropini156). Thus, the dual sugar test has been expanded into the multisugar test, which utilises additional non-fermented saccharides, typically erythritol and sucralose, for measurement of colonic permeability(Reference van Wijck, van Eijk and Buurman157,Reference Tatucu-Babet, Forsyth and Udy158) . Small intestinal permeability is assessed as per the dual sugar test, while colonic permeability is assessed by the sucralose to erythritol ratio or % sucralose recovery. Commonly urine that is collected between 5 and 24 h post ingestion is considered to be representative of the colon(Reference Seikrit, Schimpf and Wied152,Reference Wilms, Jonkers and Savelkoul153,Reference Cao, Shaw and Quarles159) , whilst other studies have used the period 8–24 h post ingestion(Reference Rao, Camilleri and Eckert160,Reference Camilleri, Nadeau and Lamsam161) . This discrepancy in protocols may be related to the variation in small intestinal transit time(Reference Fischer and Fadda162). The dual sugar, and increasingly the multi sugar, test of intestinal permeability are relatively non-invasive and cost-effective techniques for the assessment of permeability in vivo.
In addition to the above-mentioned in vivo direct permeability assessment, it is also possible to directly measure permeability ex vivo where intestinal tissue samples have been collected. These tissues may be collected as part of an endoscopic biopsy in people(Reference Vanheel, Vicario and Vanuytsel163) or following dissection in animal studies(Reference Thomson, Smart and Somerville137,Reference Clarke164) . The isolated section of intestinal tissue is mounted in an Ussing chamber and molecule probes such as PEGs or FITC-Dextran are placed on the luminal side of the tissue and flux to the basolateral side is measured to indicate epithelial permeability(Reference Thomson, Smart and Somerville137). Additionally, a small voltage may be applied across the epithelial layer and the transepithelial electrical resistance (TEER) calculated, with lower TEER values indicating lower resistance and thus increased permeability across the barrier(Reference Farré, Fiorani and Abdu Rahiman165). It is worth noting that TEER does not distinguish between the pore and leak pathways, as an increase in permeability through either pathway will lead to a reduction in TEER(Reference Vanuytsel, Tack and Farre136). The Ussing chamber technique has the benefit of being specific to the section of the intestinal tract (e.g. duodenum, ileum, sigmoid colon, etc) where permeability is observed, however, it has the disadvantage of requiring an endoscopic biopsy in studies in people.
Indirect measurements of intestinal permeability
Whilst the direct measures described above and summarised in Table 2 involve the measurement of probes that pass from the luminal to the basolateral side of the gastrointestinal epithelium, indirect measures refer to biomarkers that may relate to permeability. The presence of lipopolysaccharide, from Gram-negative bacteria, in the blood has been widely used as a marker of permeability, however, there are concerns that the limulus amoebocyte lysate assay used to measure it is not accurate at the lower levels observed with chronic low-grade inflammatory conditions(Reference Pearce, Estanislao and Fareed166). Lipopolysaccharide binding protein (LBP) is an endogenous protein that is produced in response to LPS(Reference Schumann, Leong and Flaggs167) and is commonly used as a marker of permeability. In a study comparing biomarkers with directly measured permeability, it was the only plasma biomarker to significantly correlate with the lactulose mannitol ratio, although it should be noted that the correlation coefficient ranged from r = 0·423 in healthy-weight participants to r = 0·813 in obese participants, suggesting that it may not be a highly reliable marker in all health states(Reference Seethaler, Basrai and Neyrinck154). The two other plasma markers assessed in this study, zonulin and intestinal fatty acid binding protein (I-FABP), showed no correlation with the lactulose mannitol ratio(Reference Seethaler, Basrai and Neyrinck154). Furthermore, there is evidence to suggest that commercially available zonulin kits may not be measuring zonulin, but another protein altogether(Reference Ajamian, Steer and Rosella168,Reference Scheffler, Crane and Heyne169) . An in-depth discussion regarding the plethora of purported intestinal permeability biomarkers has been published by Perez-Diaz-del-Campo et al. (Reference Perez-Diaz-Del-Campo, Castelnuovo and Ribaldone170). In summary, there is a need for more accurate biomarkers of intestinal permeability, with LBP being the current marker that appears to correlate best with directly measured permeability.
Conclusion
New technology and accessible tools will advance the identification of physiological biomarkers, enabling a better understanding of gastrointestinal function and dysfunction. Characterising motility, fermentation and permeability phenotypes that correlate with health and disease including specific disorders of gut-brain interaction will be crucial for developing targeted, individualised therapies. The ideal approaches should be non-invasive, easily applicable to large populations, highly sensitive and specific to reliably inform clinical management, and should be able to be accurately undertaken in a fed and physiological state.
Acknowledgements
The authors extend their gratitude to the attendees of the workshop titled “Design, Assessment, and Interpretation of Diet-Microbiome Interactions: Using Cutting-Edge Gut-Brain Studies as an Exemplar”, held during the 2023 combined Annual Scientific Meeting of the New Zealand and Australian Nutrition Societies, for their valuable insights and contributions.
Author contributions
M.S. conceptualised the manuscript. M.S., J.B., C.K.Y. and C.T. drafted, edited and approved the final manuscript.
Financial support
This work received no specific grant from any funding agency, commercial or not-for-profit sectors. JRB is supported by a National Health and Medical Research Council Emerging Leadership Fellowship (APP2025943). MS is supported by a National Heart Foundation Postdoctoral Fellowship (106 698). CK is supported by the Crohn’s Colitis Australia Angela McAvoy Scholarship and the International Organization for the Study of Inflammatory Bowel Diseases.
Competing interests
None.