Introduction
CPPs are potent carriers of a variety of biological cargoes into different cell types and also promising drug delivery systems (Langel, Reference Langel2019). In this respect, they were intensively studied, in the last years, particularly for introducing anticancer molecules into tumors (Zorko et al., Reference Zorko, Jones and Langel2022). Dozens of peptide-based drugs have been approved for clinical use (Zhang and Eiden, Reference Zhang and Eiden2019), but among them, CPPs could not be found. Although CPPs have been known for more than 30 years, they have not yet entered clinical practice. Altogether, around 30 CPPs are involved in clinical trials, but only a few have entered Phase III; for oncological treatment, the study of which is very intensive in the last years, only one has reached Phase I clinical trials, and no CPP or CPP-drug construct has been approved by FDA for any disease so far (Falanga et al., Reference Falanga, Lombardi, Galdiero, Genio and Galdiero2020). In this review, we present recent research summarizing studies of the composition of CPPs and their impact for carrying various cargoes into cells by biophysical methods, such as the use of fluorescence-based methods including confocal microscopy and flow cytometry, model membranes, circular dichroism (CD), nuclear magnetic resonance (NMR), and mass spectrometry (MS). The truth is that almost all methods used today in life sciences incorporate some degree of physical approach.
CPP
CPPs are short peptides of up to 40 amino acids. They can enter the cell and promote intracellular effects by themselves or by the delivery of bioactive cargoes. This definition is practically identical to its first appearance in the first book on CPPs (Langel, Reference Langel and Langel2002) and many papers on CPPs about 20 years ago (cf. Zorko and Langel, Reference Zorko and Langel2005). Many different CPPs were found in the last 30 years. According to their structures and properties, the only real common feature of CPPs seems to be their peptide nature and their ability to internalize into cells together with the cargo that is otherwise not able to reach the cell interior. The name cell-penetrating peptide and its abbreviation CPP appeared in the scientific literature first in 1998 (Pooga et al., Reference Pooga, Hällbrink, Zorko and Langel1998) and was soon generally accepted. In the literature, we can find other names for CPP, like membrane transduction peptide, Trojan horse peptide, and some others. CPPs generated from protein sequences are frequently named protein transduction domains, and peptides that interfere with processes in the cell interior by themselves are known as bioportides.
Horseradish peroxidase was the first functional protein to be carried into the cell in 1987 by using the trans-activator of transcription (Tat-protein) of the human AIDS virus (Frankel and Pabo, Reference Frankel and Pabo1988). It was shown that for the transfer of the cargo into the cell, only 11 amino acids long domain of Tat-protein is needed (Green et al., Reference Green, Ishino and Loewenstein1989), and the first CPP was born. From that time the number of CPPs increased enormously. The database of CPPs (https://webs.iiitd.edu.in/raghava/cppsite/) now (November 2021) contains almost 2000 entries with the following information: peptide sequence, nature of peptide, chemical modifications, experimental validation technique, structure of peptide, and type of cargo delivered. Analysis of the entries in this database revealed the following (Kalafatovic and Giralt, Reference Kalafatovic and Giralt2017): out of 1855 CPPs in the database, 95% of CPPs are linear and the rest 5% is cyclic; majority of the delivered cargo represent different fluorophores (54%), 15% nucleic acids, 9% proteins, 8% biotin, 7% nanoparticles, 4% peptides, and 3% other structures; majority of CPPs are composed of only standard L-amino acids (85%), 11% of CPPs consist of modified amino acids, 3% of D-amino acids only, and 2% are mixed.
Classification
Traditionally, CPPs are classified based on their physicochemical properties, origin of peptides, or other characteristic features. The physicochemical properties of CPPs arise from their basic structure. In this respect, CPPs are usually divided into three groups: cationic, amphipathic, and hydrophobic. Being composed of mostly positively charged amino acids, mainly Arg (R) and Lys (K), cationic CPPs are positively charged at physiological pH. Examples of cationic CPPs are Tat (GRKKRRQRRRPQ, Green et al., Reference Green, Ishino and Loewenstein1989), and poly-arginine (Arg)8–10 (cf. Arg8 or R8 RRRRRRRR, Futaki, Reference Futaki2006). Amphipathic CPPs are composed of a combination of hydrophilic and hydrophobic amino acids where amphipathicity is due to the sequence or is achieved after folding of the CPP into an α-helix with a hydrophilic and a hydrophobic face: an example is MAP (KLALKLALKALKAALKLA, Oehlke et al., Reference Oehlke, Scheller, Wiesner, Krause, Beyermann, Klauschenz, Melzig and Bienert1998). Hydrophobic CPPs are made of hydrophobic amino acids but some hydrophilic amino acids must also be included, otherwise, they would be insoluble in body fluids and they would also be retained in the hydrophobic core of the lipid double-layer when passing through the cell membrane. An example is Pep-7, a synthetic hydrophobic α-helix (SDLWEMMMVSLACQY, Gao et al., Reference Gao, Mao, Ditzel, Farnaes, Wirsching, Lerner and Janda2002). It should be mentioned that some anionic peptides were also shown to be able to penetrate into cells (cf. Neves-Coelho et al., Reference Neves-Coelho, Eleutério, Enguita, Neves and Castanho2017).
According to the origin, CPPs are usually also divided into three groups: protein derived, chimeric, and synthetic. Protein-derived CPPs represent a part of the natural protein structure that can be transferred through the cell membrane. The oldest example is Tat. Chimeric CPPs have two different origins, a good example is transportan (abbreviated TP, GWTLNSAGYLLGKINLKALAKISIL, Pooga et al., Reference Pooga, Hällbrink, Zorko and Langel1998), which is constructed from sequences of galanin and mastoparan. Synthetic CPPs are artificially designed, an example is MAP (see the structure above).
Another classification level, in particular, is clinically important. In this classification, CPPs are divided into cell-specific and non-cell-specific peptides. Cell-specific CPPs are able to deliver cargo into the specific cell, while non-cell-specific are not selective.
Because of the great number of very different CPPs, all levels of CPP classification are very broad and usually give overlapping results. To avoid this and to classify CPPs more precisely, taking into account as many properties as possible, a new approach was adopted. Classes of CPPs were grouped in pairs accordingly, to cover eight levels of classification. In each level, two classes of CPPs with the opposite properties were presented. This approach was recently published (Langel, Reference Langel2019) and is not further elaborated here.
Other classifications of CPPs are also available. An interesting taxonomy of CPPs, based on the mechanisms of entry into cells is given by Berkeley Kauffman et al. (Reference Berkeley Kauffman, Fuselier, He and Wimley2015).
Cell internalization
There are two main routes of CPP with or without cargo by which they can be transferred into the cells and organelles: direct penetration or one of several endocytic mechanisms. However, detailed mechanisms of CPP internalization are still not clear. It seems that direct internalization is related to the destabilization of the membrane, which is particularly true for cationic CPPs that include lysine or arginine in the structure, for instance, poly-arginines (Futaki and Nakase, Reference Futaki and Nakase2017; Murayama et al., Reference Murayama, Masuda, Afonin, Kawano, Takatani-Nakase, Ida, Takahashi, Fukuma, Ulrich and Futaki2017). Destabilization of the cell membrane can be initiated by the accumulation of positively charged CPPs on a negatively charged membrane surface, by attracting water molecules that bind to charged amino acids of CPPs into the hydrophobic core of lipid bilayers (Grasso et al., Reference Grasso, Muscat, Rebella, Morbiducci, Audenino, Danani and Deriu2018), or by inducing transfer pores in the membrane (Islam et al., Reference Islam, Sharmin, Moniruzzaman and Yamazaki2018). Membrane curvature and the depletion of the amount of membrane cholesterol could further destabilize the lipid bilayer and help peptides to traverse the membrane. For penetratin, many years ago Prochiantz (Reference Prochiantz1996) proposed that spontaneous direct translocation of the membrane proceeds by the formation of an inverted micelle. Later, Kawamoto et al. (Reference Kawamoto, Takasu, Miyakawa, Morikawa, Oda, Futaki and Nagao2011) suggested that this mechanism could be applied by several cationic CPPs.
To enter into cells with or without cargo, most CPPs employ different mechanisms of endocytosis, including clathrin- and caveolin-mediated endocytosis, other receptor-dependent endocytosis, macropinocytosis, and possibly other endocytic routes (Doherty and McMahon, Reference Doherty and McMahon2009). It seems that the most important route of internalization is micropinocytosis, particularly for cancer tissue (Yoo et al., Reference Yoo, Barros, Brown, Rabot, Bar-Sagi and Arora2020). In all these mechanisms, active target cells and energy are required. In endocytic pathways, CPPs (and cargo) almost always end up in the endosomal vesicles which further mature into the lysosomes where they are usually degraded. Therefore, fast endosomal escape is a prerequisite for the effective delivery of cargo into the cell cytoplasm and in other cell organelles. Different strategies were developed to enhance the endosomal escape of CPP and cargo to achieve more effective delivery. A review of these strategies was recently published (Pei and Buyanova, Reference Pei and Buyanova2019) but the use of some biophysical methods for the detection of endosomal escape is covered also in this review. It should be stressed here that in the delivery of cargo, many CPPs seem to be able to use both direct and endocytic routes of internalization. It was suggested for instance that Tat and penetratin can cross the membrane passively only at low concentrations while at higher concentrations, they use both direct and endocytic routes (Walrant et al., Reference Walrant, Cardon, Burlina and Sagan2017). It is interesting, that another group has obtained an entirely opposite result (Verdurmen et al., Reference Verdurmen, Mazlami and Plückthun2017). Concerning the mechanism of CPPs, the details of this feature are not very well clarified.
CPP coupling to cargo molecules
Cargo molecules or complexes should enter cells in order to act effectively. Internalization of drugs, other molecules, and nanoparticles can be highly improved by coupling to CPP.
Cell-penetrating sequences in protein structures
Concerning proteins as molecules that should enter the cell, some can penetrate cells by themselves and are also called shuttling proteins. In some cases, cell penetration of proteins can be induced or enhanced by incorporating sequences that promote cell penetration into the structure of the protein. In addition to Tat protein, several other proteins with transducing capabilities have been identified, for instance, ANTP (Joliot et al., Reference Joliot, Pernelle, Deagostini-Bazin and Prochiantz1991), HoxB4 (Amsellem et al., Reference Amsellem, Pflumio, Bardinet, Izac, Charneau, Romeo, Dubart-Kupperschmitt and Fichelson2003), the herpes simplex virus type 1 VP22 transcription factor (Elliott and O'Hare, Reference Elliott and O'Hare1997; Schwartze and Dowdy, Reference Schwartze and Dowdy2000), OCT 4 acting as self-penetrating pluripotency reprogramming factor (Harreither et al., Reference Harreither, Rydberg, Amand, Jadhav, Fliedl, Benda, Esteban, Pei, Borth, Grillari-Voglauer, Hommerding, Edenhofer, Norden and Grillari2014), homeodomain transcription factor engrailed-2, En-2 (Brunet et al., Reference Brunet, Weinl, Piper, Trembleau, Volovitch, Harris, Prochiantz and Holt2005), Engrailed 1, EN1 (Beltran et al., Reference Beltran, Graves and Blancafort2014), Knotted-1 (Tassetto et al., Reference Tassetto, Maizel, Osorio and Joliot2005), Otx2 (Bernard and Prochiantz, Reference Bernard and Prochiantz2016), Pax4 involved in vertebrate organogenesis (Lu et al., Reference Lu, Li, Lan, Zhang, Fan, Wang and Lu2007), the protein NeuroD/BETA2 (Chen et al., Reference Chen, Li, Lu, Chen, Huang, Wu, Zhang and Lu2006), protein-ligand complex neocarzinostatin, NCS (Moody et al., Reference Moody, Burlina, Martin, Morgan, Offer, Smith, Molloy and Caddick2013), omomycmini-protein, a Myc inhibitor (Massó-Valés and Soucek, Reference Massó-Vallés and Soucek2020), human papillomavirus minor capsid protein L2 (Xie et al., Reference Xie, Bi, Zhang, Dong, Teng, Lee and Yang2020a, Reference Xie, Zhang, Crite and DiMaio2020b), etc. Finding CPP sequences can be an issue of a reasonable guess according to the structure of known CPPs, or it can be generated by computer learning methods (Hälbrink et al., Reference Hällbrink, Kilk, Elmquist, Lundberg, Lindgren, Jiang, Pooga, Soomets and Langel2005; de Oliviera et al., Reference de Oliveira, Santana, Josino, Lima and de Souza de Sales Júnior2021; Rives et al., Reference Rives, Meier, Sercu, Goyal, Lin, Liu, Guo, Ott, Zitnick, Ma and Fergus2021). Successful incorporation of CPP sequence into proteins to promote effective cell penetration has been achieved in some cases. It is important not to disturb the structure and function of proteins by introducing cell penetrating sequences. Therefore, it is a usual practice to include cell penetrating sequences into the open loops (Chen and Pei, Reference Chen and Pei2020) of proteins or as flanking CPP sequences at the C-terminal or N-terminal part of the proteins. When incorporated into protein loops, CPP sequences can induce cell penetration of proteins more effectively if they form cyclic structures. Genetic engineering is of great help to express such a construct in one step. In most cases, however, molecules that should act in the cells are coupled to CPP.
Approaches to couple cargo molecules to CPPs
Cargoes can be conjugated to CPPs in different ways, but essentially by covalent coupling or by forming a complex, using multiple weak interactions (Borrelli et al., Reference Borrelli, Tornesello, Tornesello and Buonaguro2018). When covalent attachment of cargo to CPP is employed, cargo can be attached directly to CPP or via the suitable spacer where cargo is tethered to CPP through a cleavable bond, such as hydrazone or disulfide (El-Andaloussi et al., Reference El-Andaloussi, Johansson, Holm and Langel2007). It has long been known that cargo usually decreases the rate and efficiency of translocation which is dependent on the nature of the cargo and its dimension (Via et al., Reference Via, Wilke, Mayorga and Del Pópolo2020). However, in some cases, it was observed that penetration efficiency can be increased if CPP is designed to self-assemble into spherical micelles, thus locally increasing its local interface density (Weinberger et al., Reference Weinberger, Walter, MacEwan, Schmatko, Muller, Schroder, Chilkoti and Marques2017) or when the flexible spacer is replaced by a constrained cyclic spacer (Bhosle and Fernandes, Reference Bhosle and Fernandes2017). The spacer can also contribute to the selectivity of penetration of the cargo in specific, for instance, cancer, tissue (Shi et al., Reference Shi, Li, Zhang, Shen, Qi, Wang and Qi2017a, Reference Shi, Conde and Azevedo2017b). Selectivity or targeting is discussed in the next section in more detail. Oligonucleotides can also be covalently attached to CPP, either to the 3’ or 5’ site via a suitable linker, which can be cleavable or not – a recent review of these attachments of siRNA is available (Tai, Reference Tai2019). When cargo is non-covalently conjugated to CPP, the conjugation is governed by multiple electrostatic and hydrophobic interactions between CPP and cargo. An interesting example is to couple modified negatively charged leucine zipper to CPP and modified positively charged leucine zipper to a cargo molecule, or vice versa; zippers can electrostatically bind to each other thus coupling CPP to the cargo (Kitamatsu et al., Reference Kitamatsu, Yuasa, Ohtsuki and Michiue2021). Non-covalent coupling of CPP to the cargo usually results in the formation of complex nanoparticles – for review see McClorey and Banerjee (Reference McClorey and Banerjee2018).
Recently, there has been extensive research aimed toward forming liposomes and nanoparticles as delivery vehicles, decorated with CPPs for enhanced penetration into the cell. Several reviews on this topic were published (cf. Shi et al., Reference Shi, Li, Zhang, Shen, Qi, Wang and Qi2017a, Reference Shi, Conde and Azevedo2017b; Tayo, Reference Tayo2017; Gessner and Neundorf, Reference Gessner and Neundorf2020; Chiarpotti et al., Reference Chiarpotti, Longo and Del Popolo2021). Although these constructs and CPPs, in general, have not been accepted for clinical use, their potential clinical application is under intensive testing and discussion. Three most recent reviews are enlisted here (Gomes Dos Reis and Traini, Reference Gomes Dos Reis and Traini2020; Xie et al., Reference Xie, Bi, Zhang, Dong, Teng, Lee and Yang2020a, Reference Xie, Zhang, Crite and DiMaio2020b; Kurrikoff et al., Reference Kurrikoff, Vunk and Langel2021). We briefly discuss the use of fluorescence and some other biophysical methods in characterizing these structures in the next sections. Finally, an important question after cargo delivery is where the cargo is really situated, particularly in which cell compartment can it be found. A number of methods have been developed to localize cargo in the tissue and cell. A recent review of these methods has been published by Xie et al. (Reference Xie, Bi, Zhang, Dong, Teng, Lee and Yang2020a, Reference Xie, Zhang, Crite and DiMaio2020b).
Biophysical methods and CPPs
Fluorescence
Fluorescence is an extensively used approach to detect and explore CPPs. For this review, we have studied over 1200 papers dealing with CPPs that were published from 2013 to the present time and found over 450 papers in which fluorescence is the method of identification of CPPs. In the following, cell internalization of these peptides is discussed. Several review articles are available dealing with CPPs and fluorescence, we cite here only selected recent papers (Boisquérin et al., Reference Boisguérin, Deshayes, Gait, O'Donovan, Godfrey, Betts, Wood and Lebleu2015; Uusna et al., Reference Uusna, Langel and Langel2015; Barba-Bon et al., Reference Barba-Bon, Pan, Biedermann, Guo and Nau2019; Seisel et al., Reference Seisel, Pelletier, Deshayes and Boisguerin2019; Deprey and Kritzer, Reference Deprey and Kritzer2020; Knox et al., Reference Knox, Steinauer, Alpha-Cobb, Trexler, Rhoades and Schepartz2020; Sakamoto et al., Reference Sakamoto, Morishita, Aburai, Sakai, Abe, Nakase, Futaki and Sakai2020).
To apply fluorescence methods to CPPs, they should be conjugated to suitable fluorophore molecules. This means that with fluorescence, we always deal with CPP-cargo constructs. Fluorophore moiety is usually coupled to CPP by a covalent bond (see above). As it is well known, cargo can influence the behavior of CPP in many ways, particularly when it is large – some examples are given in section ‘Approaches to couple cargo molecules to CPP’. Fluorescence methods most often used are: fluorescence spectroscopy, fluorescence correlation spectroscopy (FCS), fluorescence microscopy, fluorescence confocal microscopy and confocal laser scanning microscopy, fluorescence-activated cell sorting (FACS), and, of course, fluorescent imaging. In all fluorescence methods, cells, vesicles, or other target structures are first incubated with CPP coupled to a fluorophore to allow its penetration into the cell. After a suitable time interval, the fluorophore in the target structure is excited by the absorption of light. When the fluorophore returns to the ground state, it emits light that is detected by the instrument. Each fluorophore emits light of a specific frequency. The intensity of the emitted light depends on the intensity of the excitation light and the efficiency of the fluorophore, depending on the molar extinction coefficient and quantum yield. However, light emission of the fluorophore can be modified by the fluorophore neighborhood and from these data, the interaction of the fluorophore with other molecules like proteins, peptides, or lipids can be deduced. In fluorescence spectroscopy, the bulk emitted light can be measured by a spectrofluorometer which is frequently equipped with a microplate reader to make scanning of the great number of samples faster. Pure fluorescence spectroscopy is today rarely used. By using a fluorescence microscope, the fluorescence is detected as a function of two or three-dimensional space, resolving in this way fluorescent objects in the target structure. A flow cytometer is used for the analysis of the fluorophore incorporated in the individual target cell when it flows in the liquid stream. All fluorescence-based methods have many variants and subclasses. It is a usual practice to combine these methods within fluorescence domain methods and also with other methods which do not exploit fluorescence. It seems that the best way to detect the internalization of the CPP-cargo construct into the cells and also into its target part (organelle) is the confirmation of the successful delivery of cargo by detecting the proper physiological effect of the cargo in the cell. Another approach is the co-localization of the emitted fluorescence light of the CPP delivered cargo with the light emitted or absorbed by specific target (molecule, organelle, cell type, tissue, or organ) markers. Also, potential cytotoxicity as a function of the construct concentration is checked for the safe delivery by CPP.
Due to the great number of studies that use fluorescence as a principal CPP detection method, only selected examples are presented, as follows. Some of the examples are gathered in Table 1 to enable a concise overview of these methods. The examples presented in the table do not overlap with those shown in the text.
Table 1. Selected examples of use of fluorescence methods in CPP studies
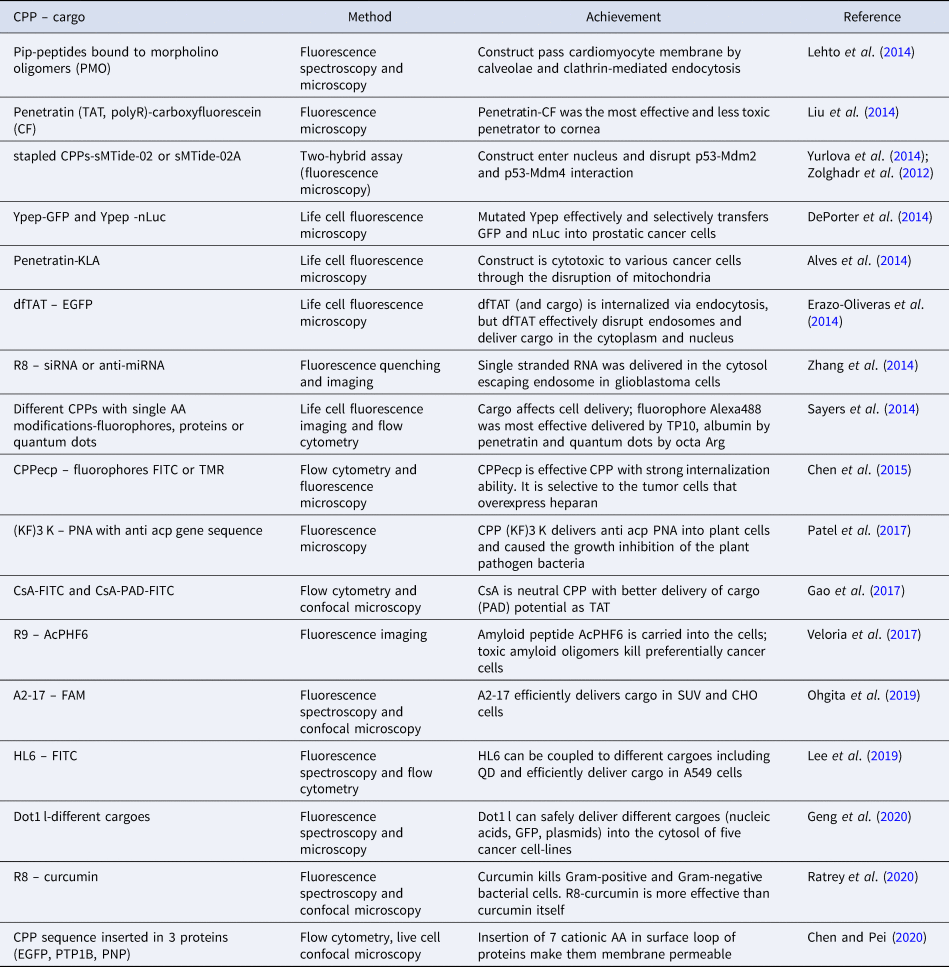
AA, amino acid; A2-17, A2, 20 AA of N-terminal domain of human apolipoprotein E, truncated by 3 AA; A549, human bronchoalveolar carcinoma cells; AcPHF6, amyloid peptide from the sequence of apolipoprotein A1; anti acp PNA, peptide nucleic acid with the antisense sequence to block mRNA of the essential bacterial gene acp; CF, carboxyfluorescein; CHO, Chinese hamster ovary cells; CPPecp, NYRWRCKNQN, heparan sulfate binding CPP; CsA, cyclosporin A, cyclic neutral CPP; Curcumin, polyphenolic natural antimicrobial compound from plant Curcuma longa; dfTAT, tetramethylrhodamine-labeled dimer of TAT; Dot-1 l, 24 AA long CPP sequence from Dot1L protein; EGFP, GFP derived from Aequorea victoria; FAM, carboxyfluorescein; FITC, fluorescein isothiocyanate; GFP, green fluorescence protein; HL6, L6, bovine lactoferricin, prolonged by 5 Hys on both termini; (KF)3 K, cationic CPP, KFFKFFKFFK; Mdm-2 and Mdm4, regulatory binding partners of p53; nLuc, NanoLuc luciferase; p53, tumor suppressor; PAD, proapoptotic domain peptide (14 AA); Pip peptides, modified hexaArg-Penetratin conjugate; PNP, purine nucleoside phosphorylase; PTP1B, human protein tyrosine phosphatase 1B; QD, quantum dot; R8, octa Arg; R9, nona Arg; sMTide-02 and sMTide-02A, stapled CPPs; SUV, small unilamellar vesicles; TAT, CPP, GRKKRRQRRRPQ; TMR, tetramethylrhodamine; TP10, transportan truncated by 6 AA; Ypep, CPP, YTLGFKTSFNVQ.
Using fluorescence microscopy and flow cytometry, the precursor of interleukin 1 alpha equipped with nuclear localization sequence (NLS) and coupled to green fluorescence protein (GFP) was shown to have CPP abilities. It penetrates the nucleus of the Jurkat and HeLa cells delivering GFP. It was also shown that it is able to deliver proteins into the cells of different mice organs (spleen, liver, intestine) after intraperitoneal administration (Koo et al., Reference Koo, Yoon, Kim, Lim, Park and Choi2014). No cytotoxicity was observed even at 100 μM concentration of the construct. Using the same combination of fluorescence methods (Wu and Gehring, Reference Wu and Gehring2014) and applying low temperature, it was shown that complete Antennapedia homeodomain protein can preferentially enter the cells via macropinocytosis. By using a combination of fluorescence methods, He et al. (Reference He, Berkeley Kauffman, Fuselier, Naveen, Voss, Hristova and Wimley2013) first identified a series of novel CPPs which were selected using synthetic membranes and termed spontaneous membrane-translocating peptides (SMPTs). These peptides were able to deliver tetramethylrodamine (TAMRA) and Alexa Fluor 546 dye into the cytoplasm of different cells under conditions in which endocytosis was prevented. When injected into mice, SMTPs delivered TAMRA in many tissues where it could be detected even after 2 h after administration while SMTP unconjugated TAMRA was rapidly cleared. They speculated that SMTPs could deliver many other polar compounds into cells. Although the mechanism of internalization of the CPP prototype TAT, which was first discovered CPP, is not clarified, it is still intensively studied. Additionally, TAT serves as a model and reference CPP, and its activity as a CPP is frequently correlated with the activity of other CPPs (cf. Kim et al., Reference Kim, Yum, Jang and Ahn2015). The cell-uptake of fluorescein isothiocyanate (FAM) labelled TAT was explored by various fluorescence methods, including fluorescence spectroscopy, flow cytometry, and confocal microscopy (Ben-Dov and Korenstein, Reference Ben-Dov and Korenstein2015). It was found that the TAT-FAM construct can enter cells independently of endocytosis by dual entry routes with different energy dependences. CPPs like TAT are intrinsically cationic peptides composed of a large number of positively charged amino acids and are prone to interact with negatively charged biological structures. There is a need for less cationic CPPs to prolong their blood circulation time and availability. This problem was addressed by the use of membrane interacting proteins as the source of the potential membrane transferring peptides. It was found that from the sequence of annexin, a number of hydrophobic CPPs can be derived (Kim et al., Reference Kim, Yum, Jang and Ahn2015) which were better cargo-delivery agents than the CPP prototype TAT, as shown by fluorescence flow cytometry and other methods. These peptides were very moderately cytotoxic, very stable in the serum, and showed no immunogenicity. An interesting method for CPP and cargo cell internalization was derived (Schmidt et al., Reference Schmidt, Adjobo-Hermans, Wallbrecher, Verdurmen, Bovee-Geurts, van Oostrum, Milletti, Enderle and Brock2015). They first transfected cells with a plasmid encoding the non-fluorescent part of GFP (GFP1-10) and used a missing part of GFP as cargo conjugated to CPP. After delivery, a missing part of GFP by CPP in the cells, both parts of GFP fused, and the fluorescence of GFP was restored. They further improved the sensitivity of the method by modification of the peptide and more effective expression. A Japanese group has found a new class of CPP, polyhistidines (PHPs), that was inspired by the fact that many CPPs are cationic (Iwasaki et al., Reference Iwasaki, Tokuda, Kotake, Okada, Takeda, Kawano and Nakayama2015). PHPs were effective CPPs; uptake was improved with the increasing number of His residues up to 16. This most effective CPP was called H16. Cell transduction and the ability to carry cargoes into the cell were studied by attaching GFP to the peptide, obtaining a GFP-H16 construct. By confocal microscopy, it was shown that the construct accumulated in the human fibrosarcoma cells and is retained in fibrosarcoma tumors for more than 5 days. A new CPP was detected and purified from silkworm hemolymph (Park et al., Reference Park, Lee, Park, Rhee, Choi and Park2012), being thus the first CPP of insect origin. It was called 30Kc19 and was later produced from recombinant Escherichia coli. Fused with GFP and using immunofluorescence microscopy, it was shown that peptide can deliver cargoes in vitro and in vivo and that it is not toxic. A problem of quantitative analysis of CPP uptake into cells is a serious problem. Combining FACS with FCS overcomes this problem, enabling precise quantification of internalized CPP-cargo constructs (Rezgui et al., Reference Rezgui, Blumer, Yeoh-Tan, Trexler and Magzoup2016). It is carried out in three steps. First, cells are incubated with a CPP-cargo construct, then cells are sorted according to CPP uptake by FACS (Bonner et al., Reference Bonner, Hulett, Sweet and Herzenberg1972), and finally, cells are lysed and the internalized peptide is quantified by FCS. Before the experiment, CPPs must be labelled with a suitable label (authors used penetratin with some modifications and fluoroAlexa Fluor 488-succinimidylester for labelling). According to the fluorescence signal, FACS collects only intact cells with internalized constructs. In the cell lysate, a precise number of fluorescently labelled molecules in the sampling volume can be determined by FCS, thus measuring intracellular fluorophore concentrations in the nanomolar range. As claimed by the authors, ‘The FACS-FCS approach provides a means for quantifying any intracellular biochemical entity, whether expressed in the cell or introduced exogenously and transported across the plasma membrane’. A French group has analyzed cell uptake of CPP-fluorescein conjugate by two methods, fluorescence spectroscopy and flow cytometry, and they correlated the obtained results with MS (MALDI-TOF) optimized internalization analysis (Illien et al., Reference Illien, Rodriguez, Amoura, Joliot, Pallerla, Cribier, Burlina and Sagan2016). They checked the ability of TAT, penetratin, and R9 CPPs to carry fluorescein into the Chinese Hamster Ovary CHO-K1 cells and obtained quantitatively and qualitatively consistent results with fluorescence spectrometry using cell lysate and MS. On the other hand, flow cytometry was less reliable due to several limitations because of fluorescence quenching; this was particularly true for the construct penetratin-fluorescein. By using doubly labelled fluorescent and biotinylated penetratin analogues, more consistent results were obtained. Fluorescence was used also to determine the role of chirality of amino acids in TAT dimer in the delivery of fluorescent cargo (tetramethylrhodamine) into HeLa, MCH58, and HDF cells (Najjar et al., Reference Najjar, Erazo-Oliveras, Brock, Wang and Pellois2016). It was shown that all D-peptides were more stable and were detected in the cytosol for several days, but all L-peptides were no more present in the cell after several hours. The ability to deliver cargo into the cell was approximately the same with both enantiomers, but a more precise observation revealed that the L-peptide was better in penetrating the cell but was less prone to escape endosomes after being internalized by the endocytic process. For the use of fluorescence methods, CPPs should be labelled with a fluorophore. It has been shown that fluorophore attachment to CPP can induce different effects on the cellular distribution of CPP (with cargo) and affects cell viability (Birch et al., Reference Birch, Vinter Christensen, Staerk, Franzyk and Morck Nielsen2017). It has been suggested that the selection of fluorophore can be critical for the results of delivery experiments. Birch et al. tested seven different fluorophores of various structures with penetratin and were able to draw the following general conclusions: fluorophore-penetratin conjugates exert strong structure-dependent reduction in viability of several mammalian cell types as compared to non-labelled penetratin; effects on membrane integrity, as well as intracellular distribution patterns, differed among the conjugates; neutral hydrophobic fluorophores or negatively charged fluorophores conferred less cytotoxicity as compared to the effect exerted by positively charged, hydrophobic fluorophores. To improve the detection of cell uptake of CPPs in the cytosol, two β-lactamase fluorogenic assays were developed (Ston et al., Reference Ston, Heinrich, Juraja, Satiaputra, Hall, Anastasas, Mills, Chamberlain, Winslow, Priebatsch, Cunningham, Hoffmann and Milech2018). In one assay, a complete functional enzyme β-lactamase is delivered into cells by CPP. Then cells are incubated with dye CCF2-AM, which rapidly enters the cells, and when in the cytosol, it is converted to CCF2 by endogenous esterases. CCF2 is a negatively charged fluorescence resonance energy transfer (FRET) incorporating substrate of β-lactamase and is retained in the cytosol. If this enzyme is delivered to the cytosol by CPP, it cleaves FRET. This results in the change of fluorescence from green to blue and the presence of β-lactamase in the cytosol is confirmed. The second assay is the variant of the first one based on the splitting of β-lactamase into two non-functional components. One component is expressed in the cell and another is carried into the cell by CPP. Both components are fused in the cell cytosol and the enzyme becomes functional.
Entrance of viruses into cells is mediated by their capsid proteins. Therefore, virus capsid proteins are potential sources of new CPPs. It was observed (Yu et al., Reference Yu, Zhan, Xue, Dong, Wang, Jiang, Wang, Sun and Yang2018) that porcine circovirus type 2 has a single capsid protein Cap, which contains a nuclear localization signal sequence (NLS) incorporating also several positively charged amino acid residues. This makes this sequence a potential CPP. NLS of the virus was synthesized and was named NLS-A. Its ability to carry cargo into cells was tested using flow cytometry and confocal laser scanning microscopy. For this purpose, NLS-A-EGFP and NLS-A-FITC constructs were developed. As it was shown, NLS-A was rapidly transferred into cells via direct translocation of the peptide. That occurred after the accumulation of NLS-A on the cell membrane surface what resulted in the increased membrane permeability. After entering the cell, NLS-A was found to be evenly present in all components of the cell.
As explained in many review articles on CPPs, first, it was thought that CPPs can enter unselectively in all types of cells and all tissues. As drug delivery vehicles, it is desired for CPPs to be selective and to deliver cargo into specific types of cells or in specific organelles, particularly when they are designed to carry anticancer drugs into tumor cells (Zorko et al., Reference Zorko, Jones and Langel2022). Here we present a design of CPPs targeting the cell nucleus and subnuclear region, as confirmed by confocal scanning system and flow cytometry (Gronewold et al., Reference Gronewold, Horn, Ranđelović, Tóvári, Muñoz Vázquez, Schomäcker and Neundorf2016). Peptide N50 with the NLS and NrTP, a peptide with a nuclear targeting sequence, were coupled to the shortened CPP sC18, obtaining thus two novel peptides N50-sC18 and NrTP-sC18. It was shown that these two peptides function as efficient vectors for different cargo delivery into the nucleus and nucleoli. No toxicity of these peptides up to 100 μM concentration was observed. As it was shown, muscle ischemia can arise from mutations in the genes encoding dystrophin protein, possibly leading to different types of muscular dystrophy. In particular, mutations in the region that encodes dystrophin binding domains for nitric oxide synthase (nNOS) promote loss of nNOS from the sarcolemma, resulting in non-functional muscles. Some efforts were undertaken to reverse this pathological state of muscle by use of CPP and by control via fluorescence methods (Zhao et al., Reference Zhao, Yang, Wasala, Zhang, Yue, Duan and Lai2019). They first identified the dystrophin sequence responsible for binding to nNOS and then constructed a peptide with this sequence (called R16/17) fused to GFP for fluorescence detection and different CPPs for cell internalization. Out of several CPPs tested, TAT showed the best internalization abilities. Construct TAT-R16/17-GFP successfully entered impaired muscle cells, partly restored muscle force, and improved muscle perfusion during contraction. Many times, in fighting diseases, this can be achieved by introducing antibodies into the cell or even into the specific cell compartment. This can be done by binding the antibody to CPP. However, to retain antibody functionality, it is important to know where the transporting peptide is attached to the antibody. This was first explored by genetically fusing five selected CPPs to different positions of IgG in order to transport IgG into the cytosol of the target cancer cells. Authors selected six positions in the structure of IgG for the attachment of CPP: N and C terminals of the light chain, N and C terminals of the heavy chain, and two positions inside the heavy chain, one before the -SS- bridges in the hinge and the other after the -SS- bridges. To report the exact position of the internalized antibody in the cell, GFP was attached to CPP in the IgG-CPP construct. It was shown that two CPPs, Pep-1 (Morris et al., Reference Morris, Depollier, Mery, Heitz and Divita2001) and PEPth (Arrouss et al., Reference Arrouss, Nemati, Roncal, Wislez, Dorgham, Vallerand, Rabbe, Karboul, Carlotti, Bravo, Mazier, Decaudin and Rebollo2013) attached into the heavy chain of the antibody before the hinge was able to transfer functional IgG into the cytosol of three types of colon cancer cells in concentrations around 10% of that outside of the cells. Recently, CPP promoted the delivery of antibodies into cells. This was also studied by other groups that monitored the process by fluorescence. Sauter et al. (Reference Sauter, Strieker, Kleist, Wischnjow, Daniel, Altmann, Haberkorn and Mier2020) attached eight different tetrameric variants of CPP to the after-hinge part of the heavy chain of a monoclonal antibody, out of which TAT and penetratin were very effective transfer vehicles. Another study of the antibody-CPP construct was provided by Chong et al. (Reference Chong, Oh, Min, Park, Choi, Ahn, Chun, Lee, Yu and Lee2021) who non-covalently bound IgG (against NF-κB) with domain Z (affibody to bind antibodies) decorated with dimeric or tetrameric LK-1 CPP (LK-1 is cationic CPP composed of 16 amino acids out of which 14 represent Arg and Lys). These constructs entered the cells using ATP-dependent endocytosis. Anti-NF-κB IgG was functional in the cytosol which was accessed after internalization and successful escape from endosomes. They also provided a cleavable link that released antibodies from CPP after reaching the cytosol. Finally, we present here a study of Jakka et al. (Reference Jakka, Govindaraj and Mugesh2019) in which they showed that a very small change of one hydrogen atom in Tyr-39 of GFP for the halogen (they used Cl, Br, or I) can facilitate the membrane transport of GFP in mammalian cells. They also claimed that such a strategy can be used to improve cell penetration of small molecules (including CPPs) into cells. This example is included because GFP is frequently used as a reporter molecule to detect the internalization of different CPP constructs into cells. Proteins can be made membrane-permeable also by incorporating a CPP sequence into their open loops (see Table 1 and section ‘Cell-penetrating sequences in protein structure’).
There is a huge number of different small particles like quantum dots, nanoparticles, co-polymer structures, liposomes, and micelles that are used or tested for drug delivery. Many of these structures are decorated with CPPs to enhance their cell penetration. Composition, size, cell-delivery potential, and other characteristics of these structures are determined with biophysical methods. However, this is a specific topic that deserves a separate presentation and will be here only briefly described. As for fluorescence use, quantum dots behave as fluorophores able to absorb and consequently emit light. It has been shown that by coupling CPPs to quantum dots, these structures can be efficiently carried into cells and can be detected by fluorescence microscopy (Farkhani et al., Reference Farkhani, Johari-ahar, Zakeri-Milani, Mojarrad and Valizadeh2016). Other small particles should be labelled with fluorophores to enable fluorescent detection and imaging. Liposomes are small closed membrane particles in which interior solutions can be loaded with different cargoes. Recently, liposomes were extensively used to deliver parts of DNA, RNA, or other structures into cells. They are able to deliver cargo, for instance, siRNA, by fusing with the cell membrane spontaneously (Alshehri et al., Reference Alshehri, Grabowska and Stolnik2018), but are much better cargo deliverers when decorated with CPP. For this purpose, different CPPs can be used, most frequently TAT (cf. Bartomeu Garcia et al., Reference Bartomeu Garcia, Shi and Webster2017), polyarginines (cf. Wu et al., Reference Wu, Huang, Wang, Chen, Mi, Ying, Wang, Zhao and Huang2018), and many others. Micelles have a hydrophobic interior and are usually used to deliver non-polar molecules, many times cytostatic. An example is the delivery of the cytostatic paclitaxel (PTX) into model breast cancer cells or organisms (Shuai et al., Reference Shuai, Cai, Zhao and Sun2020). Many times, CPP molecules polymerize or form larger spherical structures by non-covalent bonding when they are mixed with cargo molecules, particularly fragments of nucleic acids. These molecules are negatively charged and form electrostatic interactions with cationic CPPs, but other combinations are also known. An example is the roughly spherical complex between pDNA and fluorescein labelled nona-arginine which entered the cell and was internalized into the nucleus, as revealed by fluorescence imaging (Oba et al., Reference Oba, Demizu, Yamashita, Kurihara and Tanaka2015). Nanoparticles are not very strictly defined and can represent very different small particles (smaller than 500 nm in diameter, but the definition is not precise). Therefore, some overlapping can be encountered particularly with small complexes described above. In spite of this, the term nanoparticle is widely used. A good example of this is WRAP-siRNA nanoparticles introduced by Deshayes et al. (Reference Deshayes, Konate, Dussot, Chavey, Vaissière, Van, Aldrian, Padari, Pooga, Vivès and Boisguérin2020). They developed amphipathic CPPs called WRAP that can form a complex with siRNA which they called nanoparticles. WRAP successfully delivered siRNA into the cells of several different cell lines mainly by direct cell membrane translocation directed by membrane destabilization, but also by endocytic pathways. Internalization was observed by fluorescence spectroscopy and microscopy. Golden, silver, and other metallic nanoparticles are also known and they were decorated by CPPs to enhance adsorbed drug delivery (Riveros et al., Reference Riveros, Eggeling, Riquelme, Adura, Loez-Inglesias, Guzman, Araya, Almada, Juarez, Valdez, Fuentevilla, Lopez and Kogan2020; Zucker and Boyes, Reference Zucker and Boyes2020). An overview of nanoparticles behavior in in vivo systems is given in Lane et al. (Reference Lane, Qian, Smith and Nie2015); Chiarpotti et al. (Reference Chiarpotti, Longo and Del Popolo2021); and Gessner and Neundorf (Reference Gessner and Neundorf2020).
Radioactivity
Instead of fluorophores, CPPs can be labelled for visualization in cells also with other markers. One approach is radioactive labelling. In a typical experiment, cells are first incubated with labelled CPP. Afterwards, cells are washed with a solution that contains a suitable diluted acid to remove the labelled CPP which is adsorbed on the outer side of the membrane. Then radioactivity is determined by the radioactivity counter and the amount of internalized CPP is calculated. If the incubation of the cells is terminated after different time intervals, also, the kinetics of the CPP internalization can be followed. Different radioactive nuclides have been used for labelling CPPs and are presented in the following examples:
One of the first examples of radioactively labelled CPP is TP labelled with 125I (Pooga et al., Reference Pooga, Hällbrink, Zorko and Langel1998). The amount of internalized TP into Bowes cells could be determined as a function of time and in this way also, the kinetics of the labelled TP internalization was followed. A drawback of this method is the inability to discriminate between intact radioactive CPP and its radioactive products after the cleavage in the cell. A more recent example is using 125I to label four cationic CPPs TAT, octa- and nona-arginines, and MAP, and follow their internalization into CHO cells (Zaro et al., Reference Zaro, Vekich, Tran and Shen2009). Another group of proteins labelled with 125I involved in the metabolism of arginine can be fused to CPPs to observe the internalization of the construct in HeLa and breast cancer cells (Yeh et al., Reference Yeh, Chen, Chen, Shen, Ann, Zaro and Shen2016). The first of these two studies revealed that MAP was able to penetrate the nuclear membrane while TAT and poly-arginines were mainly retained in the cytosol. It should be stressed here that labelling with 125I requires the presence of Tyr in the peptide structure; if this is not the case, the sequence of the peptide might be prolonged with Tyr, but its putative effect on the CPP behavior should be tested separately. In the study undertaken by Zaro et al. (Reference Zaro, Vekich, Tran and Shen2009), this was done with poly-arginines. Other nuclides recently used in radioactivity studies following CPP internalization were 68Ga (Gronewold et al., Reference Gronewold, Horn, Ranđelović, Tóvári, Muñoz Vázquez, Schomäcker and Neundorf2016), and 64Cu (Bergmann et al., Reference Bergmann, Splith, Pietzsch, Bachmann and Neundorff2017; Henry et al., Reference Henry, Chaney, Nagle, Cropper, Mozaffari, Slaybaugh, Parang, Andreev, Reshetnyak, James and Lewis2020). Recently, a review was published (Gharibkandi et al., Reference Gharibkandi, Conlon and Hosseinimehr2020) which does not deal specifically with CPPs but is generally aimed at the radioactive labelling of peptides and shows additional nuclides that could be used for the radioactive labelling of CPPs: 99mTc, 111In, and 177Lu.
Mass spectrometry
To test molecules using MS, they must first be ionized or coupled to an ionic marker. An ionic molecule or its fragments pass through an electromagnetic field where they are separated according to the mass/charge ratio which is then revealed by a mass spectrum. In the field of CPPs, this is mainly used to confirm the proper synthesis of peptides – the calculated mass of the peptide is compared to the mass determined by a mass spectrometer. Ionization of the tested compounds can be achieved in the instrument by application of a high temperature, by an electric field, or by a beam of electrons, ions, or photons. Many biological molecules are very sensitive and should not be affected by high energy impact. For these, MALDI-TOF spectrometry is more suitable. MALDI means matrix-assisted laser desorption/ionization, and TOF is a time-of-flight mass analyzer. In this instrument, the tested molecule is adsorbed in the target plate of the instrument with matrix molecules that are heated by a laser beam. After this, the matrix molecules are energized and carry tested molecules through the electromagnetic field; during this process, the tested molecules are ionized, usually into one positively charged ion. Also, in this process, the tested molecules are much more softly treated than in the classical mass spectrometer; therefore, MALDI-TOF is appropriate for biological materials. Mass spectrometric methods are considered qualitative, but they can be used also for the quantitative determination of cell-internalized CPPs if those are labelled by isotopes, and particularly when the method is combined with chromatography, usually with high-pressure liquid chromatography. One of the first studies of CPP with MS was cellular uptake of pVEC and its all-D analogue (Elmquist and Langel, Reference Elmquist and Langel2003). As it was shown by the MALDI-TOF approach, both peptides were internalized in aortic endothelial cells and murine fibroblasts, but only the all-D analogue remained intact in the cells while the all-L analogue was enzymatically decomposed. Later, several studies revealed that all-D analogues of CPPs remain stable in cells for a long time (cf. Youngblood et al., Reference Youngblood, Hatlevig, Hassinger, Iversen and Moulton2007). MALDI-TOF was used (Burlina et al., Reference Burlina, Sagan, Bolbach and Chassaing2005) to determine cell internalization of different biotinylated CPPs labelled with stable isotopes (isotope was used as an internal standard). They could also follow the CPP degradation. More recently, MALDI MS was used to observe proper targeting of the brain by nanostructures composed of CPP TAT and P42 peptides (peptide derived from human Huntingtin protein) after per mucosal administration (Arribat et al., Reference Arribat, Talmat-Amar, Paucard, Lesport, Bonneaud, Bauer, Bec, Parmentier, Benigno, Larroque, Maurel and Maschat2014). Selenium labelled penetratin was studied by electrospray MS. The study revealed massive degradation of the construct after internalization into HeLa cells (Möller et al., Reference Möller, Gabel-Jensen, Franzyk, Bahnsen, Stürup and Gammelgaard2014). By a similar approach, the degradation of TP10 CPP was also studied, showing that for the stability of the peptide Lys in the C-terminal part of the peptide is very important and also for the penetration into cells (Xue et al., Reference Xue, Liu, Wang and Zu2015). MALDI-TOF was used also for the observation of the inhibition of histone demethylase by TAT conjugated demethylase inhibitor that was able to penetrate the nucleus (Dorosz et al., Reference Dorosz, Olsen, Teuber Seger, Steinhauer, Bouras, Helgstrand, Wiuf and Gajhede2017).
Electron microscopy
Electron microscopy has been used for a very long time to study biological structures and processes (cf. Scott and Parker, Reference Scott and Parker1939). In principle, the electron microscope functions like any other microscope, only that instead of photons, it uses electrons. A beam of electrons is generated, focused by electron magnetic lenses, and accelerated by high voltage. The targeted sample material must be very thin and electrons pass through the sample where the electrons are scattered. According to the density of the different parts of the sample, the corresponding picture of the sample is obtained which can be refined by ocular electronic lenses. Two basic types of instruments with many variations are in use, transmission electron microscope (TEM) and scanning electron microscope (SEM). In TEM, electrons pass through the sample and the obtained picture can reveal the inner structure of the sample, for instance, the cell. In SEM, electrons will not pass through the sample but will induce a secondary beam of electrons that arises from the surface of the sample. In this way, a precise image of the surface is obtained. Both TEM and SEM types of electron microscopy are in use for studying CPPs.
Using TEM, one can explore biological membranes, organelles, nanoparticles, supramolecular complexes, and even bigger macromolecules, but the resolution does not permit direct visualization of a single CPP. For this, CPP must be labelled with an electron-dense tag like gold, iron oxide, and silica nanoparticles or are visible when coupled to big proteins (Padari et al., Reference Padari, Säälik, Hansen, Koppel, Raid, Langel and Pooga2005; Säälik et al., Reference Säälik, Padari, Niinep, Lorents, Hansen, Jokitalo, Langel and Pooga2009; Margus et al., Reference Margus, Padari and Pooga2013). Alternatively, a label can also be put on cargo. This has been used with oligonucleotide cargoes that were usually labelled with colloidal gold particles or quantum dots (Arukuusk et al., Reference Arukuusk, Pärnaste, Oskolkov, Copolovici, Margus, Padari, Möll, Maslovskaja, Tegova, Kivi, Tover, Pooga, Ustav and Langel2013; Margus et al., Reference Margus, Juks and Pooga2015). Very rarely were TEM observations used as the single detection method, but was frequently combined with other biophysical and biochemical methods. Such an example is also the study of extracellular vesicles that were decorated by different arginine-containing CPPs (from 4 to 16 Arg) which make internalization possible (Nakase et al., Reference Nakase, Noguchi, Aoki, Takatani-Nakase, Fujii and Futaki2017). TEM was here used to explore (together with some other methods) the shape, dimensions, and consistency of vesicles, while internalization was followed by fluorescence. As it was found, internalization of vesicles was dependent on the amount of Arg residues in CPPs and peptides with 16 Arg residues as the most effective uptake vehicle. Similarly, TEM was used for the characterization of the morphology and size of liposomes conjugated to CPP Pep-1, but internalization studies were undertaken by fluorescence (Jiao et al., Reference Jiao, Li, Pang, Zheng and Zhao2017). On the other part, crotamine conjugated gold nanoparticles additionally decorated with polyethylene glycol were internalized in the HeLa cells which was followed by TEM (Karpel et al., Reference Karpel, da Silva Liberato, Campeiro, Bergeon, Szychowski, Butler, Marino, Cusic, Goncalves de Oliveira, Oliveira, de Farias, Villares Portugal, Andrade Alves, Daniel and Hayashi2018). A variant of TEM, cryo-TEM was used to follow penetratin conjugated liposomes crossing the blood–brain barrier, accumulated in vivo in the rat brain (Tremmel et al., Reference Tremmel, Uhl, Helm, Wupperfeld, Sauter, Mier, Stremmel, Hofhaus and Fricker2016).
The SEM was also recently used for studying CPP mechanisms, usually in combination with other methods. An example is liposome treated with transferrin and CPP (penetratin and Tat) with encapsulating cytostatic doxorubicin that was able to penetrate the blood–brain barrier and deliver doxorubicin in vitro and in vivo (Sharma et al., Reference Sharma, Lakkadwala, Modgil and Singh2016). A combination of fluorescence microscopy and SEM revealed the modified membrane and morphology of the cells of five harmful microorganisms after interaction with CPP P-7; however, P-7 did not lyse the cells (Li et al., Reference Li, Shi, Cheng, Xia, Jepkorir Cheserek and Le2014). The antibacterial effect of this CPP was also the result of its interaction with microorganism DNA after uptake into the microorganism cytoplasm. A peptide designed to have cell penetration and antimicrobial properties was designed and tested with Escherichia coli (Alaybeyoglu et al., Reference Alaybeyoglu, Gedik Uluocak, Sariyar Akbulut and Ozkirimli2017). The antimicrobial effect of the peptide was monitored with fluorescence and SEM. Using TEM, SEM, and other methods, the effect of the modified peptide CADY-K (all-D retro sequence), forming nanoparticles that were able to deliver siRNA into cells, was studied (Vaissiére et al., Reference Vaissière, ldrian, Konate, Lindberg, Jourdan, Telmar, Seisel, Fernandez, Viguier, Genevois, Couillaud, Boisguerin and Deshayes2017). It was proven that the peptide has good antibacterial properties also because it is an effective CPP.
Circular dichroism
CD is a method that is based on differences of absorption of left- and right-handed circularly polarized light by chiral molecules. Regarding biomolecules, it is very useful for the determination of the secondary structure of peptides and proteins, but nucleic acids can also be analyzed. In proteins and peptides, light is mainly absorbed by the π-electrons of the atoms involved in peptide bonds. Each type of the secondary structure of peptides and proteins shows different spectra in the interval between 190 and 250 nm. The result is usually expressed in a relative proportion of α-helix, β-structure, and a random coil of the tested protein or peptide. Changes in the environment of the tested molecule are reflected in changes in the secondary structure and can be regularly monitored by CD spectrometers. Factors that affect CD spectrum are temperature, composition of the solution (solvent polarity, presence of ions, their composition, and concentration, etc.), and specific binding to different structures (receptors, lipids, and in the case of CPP different cargoes). Therefore, the conditions in which measurement is undertaken should be carefully monitored. A review of the physical principles of CD spectroscopy has been recently published (Andrews and Tretton, Reference Andrews and Tretton2020).
It is almost a routine to determine the secondary structure of every new invented CPP, in relation to cargo delivery (Vasconcelos et al., Reference Vasconcelos, Madani, Arukuusk, Pärnaste, Gräslund and Langel2014). CD spectra are usually determined in water solution and in solutions containing phospholipid vesicles with the aim to find differences that would help to explain the mechanism by which CPP passes the lipid bilayer. It seems that no specific secondary structure exists that would be common to all CPPs and would ensure passage through the membrane; uptake into the cell was obtained with peptides that adopted an α-helical, β-sheet, or a random coil structure. In spite of this, it is sometimes suggested that peptides with some specific structure, most often α-helical structure, have some advantage in passing the lipid bilayer. This arises from the early determination of the secondary structure of the first known CPPs. Even before short TAT peptide was declared as a CPP, different parts of its parent protein has been inspected for the secondary structure in a vacuum and it was found that the fragment Tat(38–60), in particular, is able to adopt the α-helical structure and a shorter part of it, Tat(38–45) forms an amphipathic helix (Loret et al., Reference Loret, Vives, Ho, Rochat, van Rietschoten and Johnson1991). Other parts of Tat protein (Tat(65–81), Tat(32–48) and Tat(32–72)) were shown to be very flexible and can adjust their conformation to be able to bind to specific binding partners (Chen and Frankel, Reference Chen and Frankel1995; Metzger et al., Reference Metzger, Schindler, Willbold, Kraft, Steegborn, Volkmann, Frank and Rösch1996). Another early confirmation study was done with penetratin and its analogues, for which, particularly for pAntp (43–58), it was shown that it can form an amphipathic helical structure, but it could also form aggregates in a 2(7) ribbon conformation (Berlose et al., Reference Berlose, Convert, Derossi, Brunissen and Chassaing1996). Finally, CD studies revealed that transportan has random coil secondary structure in water and can adopt up to 60% helical structure in SDS micelles. This was confirmed with transportan and penetratin that when being transferred from water to a solution containing different phospholipid vesicles, these two CPPs can be up to 60% helical (Magzoub et al., Reference Magzoub, Kilk, Eriksson, Langel and Gräslund2001).
Many recent studies of the secondary structure of CPPs were focused on arginine-rich CPPs. By one such study (Patil et al., Reference Patil, Naik, Vij, Yadav, Kumar, Ganguli and Fernandes2014) was shown that arginine-reach CPPs (R-X-R)4 with constrained amino acids in position X are improved cellular transporters because of the constraints introduced by the insertion of the proline-derived spacer. It was also shown that spacers keep peptides in a helical conformation and cell penetration ability is strongly influenced by the combination of hydrophobicity and flexibility of the peptide, which after internalization could be found in the cytoplasm. Another group has explored seven arginine-rich CPPs which were analogues of RW9 (RRWWRRWRR) by replacing Trp with Phe, measuring CD spectra, and assessing their penetration ability (Jobin et al., Reference Jobin, Blanchet, Henry, Chaignepain, Manigand, Castano, Lecomte, Burlina, Sagan and Alves2015). In contact with the membrane, all peptides were structured, mainly in a helical conformation. This means that the replacement of Trp with Phe did not have much influence on the propensity of the peptides to be in a helical form, but it drastically reduced uptake into the cells and made the interaction of CPP with the membrane stronger. In another very recent study (Ohgita et al., Reference Ohgita, Takechi-Haraya, Okada, Matsui, Takeuchi, Saito, Nishitsuji, Uchimura, Kawano, Hasegawa, Sakai-Kato, Akaji, Izutsu and Saito2020), arginine-rich CPP was fused to a polyproline, which introduced a more stable helical structure and better uptake into CHO cells. A study of the artificial cationic CPP KL4 was undertaken (Qiu et al., Reference Qiu, Lo, Kwok, Mason and Lam2020) in which hydrophobic amino acid residues of leucine were replaced by alanine or valine in order to obtain a more soluble peptide. It has been observed by CD that this replacement shifted the secondary structure of the peptide from predominantly helical to a more disordered structure and also decreased the peptide's ability for internalization. All these studies fuel the opinion that the helical or at least ordered structure of cationic CPPs enhanced their ability to penetrate cells. The biological application of polypeptides with ordered secondary structures is discussed in more detail in the review (Ge et al., Reference Ge, Ye, Wu, Zhu, Song, Liu and Yin2020). These and similar observations raised the idea to stabilize the helix structure in CPPs to improve cargo delivery into cells. This was achieved mainly by introducing stapled CPPs in which the helical structure is fixed by inserting a staple spacer in the peptide structure which would decrease peptide flexibility and keep the peptide in the helical form. An early attempt of more stable helical CPPs was made by making seven types of peptides with three L-Arg and up to six L-Leu (Kato et al., Reference Kato, Oba, Nishida and Tanaka2014) in which 1-aminocyclopentane-1-carboxylic acid was introduced. This stabilized the helical structure of the peptide and also improved the internalization potential of the peptides, however, without stapling. The best cargo deliverers were peptides composed of an equal number of Arg, Leu, and 1-aminocyclopentane-1-carboxylic acid; this peptide was retained in 310/α-helical conformation. Another strategy to obtain helical CPPs is to synthesize topologically constrained amphipathic peptides that are unordered in solution but form a very stable α-helix when in contact with the membrane (Jerath et al., Reference Jerath, Goyal, Trivedi, Santhoshkumar and Ramakrishnan2020). These peptides are also analyzed by CD and with complementary methods. They are effective deliverers of methotrexate in cancer cells and are much less toxic to normal cells. Real staple CPPs were described in the study by Tian et al. (Reference Tian, Jiang, Li, Wang, Zhao and Li2017) who synthesized a series of stapled peptides with different types of cross-links and comparatively studied their secondary structure, stability, and internalization potential. All synthesized peptides were able to enter the cells; however, their potential to deliver cargo into the cell was better correlated with their hydrophobicity than with their ability to form a stable helix. In the study of Hyun et al. (Reference Hyun, Choi, Neul Lee, Lee, Oh, Lee, Lee, Lee and Yu2018) a number of stapled CPPs were synthesized. All were stapled and contained various amounts of Lys and Leu residues. One of these peptides (stEK) was a deliverer of siRNA in nanomolar concentration and was further modified by replacing several Lys residues with His (it was named LKH-stEK). LKH-stEK was internalized via endocytosis but was able to escape from endosomes and successfully delivered siRNA into the cytosol. At least two review articles were recently published, dealing with stabilized and stapled peptides (Fominaya et al., Reference Fominaya, Bravo and Rebollo2015; Li et al., Reference Li, Chen, Zhang and Hu2020).
Cycling CPPs are another type of CPPs characterized by enhanced stability and improved delivery potential. Models for these peptides are several natural cyclic peptides that are present in organisms and show remarkable stability. One reason for their stability is the fact that they do not have N- and C-terminals and thus are not substrates of exopeptidases. Cycles can be introduced into the peptides by different approaches. One, rather simple approach, is to use Cys residues to make a disulfide junction. An example of this approach is the study of D'Souza et al. (Reference D'Souza, Troeira Henriques, Wang and Craik2014). They tested two ultra-stable disulfide-rich peptides, trypsin inhibitors from plants (MCoTI-II and SFTI-1). They are both good cargo deliverers but this ability can be further improved in MCoTI-II by introducing a higher number of positive charges into their structure, while mutation of SFTI-1 was not effective. A similar approach was used by Oba et al. (Reference Oba, Kunitake, Kato, Ueda and Tanaka2017). They inserted two cyclic α,α-disubstituted α-amino acids into an L-Arg nonapeptide (R9 peptide). Then the stapled peptide was provided by the incorporation of a staple into the side chain of the unstapled peptide. The modified peptide formed a stable helical structure with an enhanced uptake into the cell. Another group has coupled TAT to GFP in two ways (Nischan et al., Reference Nischan, Herce, Natale, Bohlke, Budisa, Cardoso and Hackenberger2015). One obtained construct was linear and the other was made cyclic by using azido-functionalized TAT and alkyne functionalized GFP. The cyclic construct delivered GFP into the cytosol and nucleus, but the linear construct was not detected – it was probably degraded in endosomes.
Nuclear magnetic resonance
NMR spectroscopy is a powerful spectroscopic method that uses the magnetic properties of atomic nuclei. These behave like small magnets that can absorb energy from the surrounding magnetic field provided by the strong magnets of the NMR equipment. The absorbed magnetic energy, which can be measured, depends on the close environment of the atom, particularly on the magnetic properties of atomic nuclei in the vicinity. On this ground, distances between atomic nuclei of the sample can be assessed and the structure of the sample molecule can be determined. For NMR spectroscopy, the most valuable are atoms with an uneven number of protons and neutrons in the nucleus, such as 1H, 19F, and isotopes, such as 13C, 15N, 31P, etc., because they have the best magnetic properties. NMR can provide important information on the structure of a biomolecule, such as proteins, nucleic acids, carbohydrates, and lipids. Besides, it can also reveal the interactions between them. In the field of CPPs, most NMR studies were dealing with the interaction between CPPs and the lipid bilayer. An important advantage of the method is that it can provide the structure of biomolecules in solution, but its drawback is that it can be used only for the structure determination of relatively small molecules (up to 50.000 Daltons, even if the NMR active isotopes are incorporated in sample molecules).
The first NMR studies were undertaken with fragments of Tat protein even before part of this protein was shown to be TAT CPP. The potential of the 25 amino acids long fragment of Tat protein to form α-helical structure was revealed by CD and NMR (Mujeeb et al., Reference Mujeeb, Bishop, Peterlin, Turck, Parslow and James1994) and the interaction of Tat(32–72) with RNA was shown (Metzger et al., Reference Metzger, Bayer, Willbold, Hoffmann, Frank, Goody and Rösch1997). Later, it was shown that TAT CPP induces perturbations in anionic lipid (Ziegler et al., Reference Ziegler, Blatter, Seelig and Seelig2003). Penetratin was studied in a nonpolar environment and its behavior toward lipids was addressed. In SDS micelles, penetratin adopts a helical structure (Lindberg and Gräslund, Reference Lindberg and Gräslund2001) but also in phospholipid bicelles where it is located near the surface; its less polar analogue behaved very similarly (Lindberg et al., Reference Lindberg, Biverstahl, Graslund and Maler2003). It was also shown that penetratin is not able to cross an artificial phospholipid membrane (Drin et al., Reference Drin, Mazel, Clair, Mathieu, Kaczorek and Temsamani2001) and that its structure incorporates a bent helical structure (Czajlik et al., Reference Czajlik, Mesko, Penke and Perczel2002) that disappears in a more polar environment. Helical structure and ability to penetrate the cells are not correlated (Letoha et al., Reference Letoha, Gaal, Somlai, Czajlik, Perczel and Penke2003). Penetratin was also able to disturb a lipid membrane and induce it to be negatively curved (Lamaziere et al., Reference Lamaziere, Wolf, Lambert, Chassaing, Trugnan and Ayala-Sanmartin2008). A number of CPPs were explored in SDS micelles and the phospholipid environment and it was shown that for Pep-1, some of its modifications, R9, Engrailed-2, HoxA-13, and Knotted-1 were able to form stable helices in this environment (Weller et al., Reference Weller, Lauber, Lerch, Renaud, Merkle and Zerbe2005; Balayssac et al., Reference Balayssac, Burlina, Convert, Bolbach, Chassaing and Lequin2006).
There are several other examples of exploring the interaction of CPPs with lipids in membrane bilayers, vesicles, or micelles, practically all showing the induction of the helical structure of CPPs in contact with lipids, but they are not presented here. More recent experiments are as follows. Protein Epsin-1 and its derived 18 amino acids long peptide are able to induce positive curvature in the membrane spots where clathrin-coated pits arise. This positive curvature of the membrane is the spot that is found by polyarginine CPPs and enhances their penetration into the cell (Pujals et al., Reference Pujals, Miyamae, Afonin, Murayama, Hirose, Nakase, Taniuchi, Umeda, Sakamoto, Ulrich and Futaki2014). For BP100, a short 11 amino acid long peptide, it was shown that it obtained α-helical structure interacting with different artificial, prokaryotic, or eukaryotic membranes and induced substantial thinning of the membrane, which was shown to be a prerequisite for penetration into cells (Misiewiz et al., Reference Misiewitz, Afonin, Grage, van den Berg, Strandberg, Wadhwani and Ulrich2015). A group produced the peptide EETI-II, a model Cys-knot peptide (Gao et al., Reference Gao, Stanger, Kaluarachchi, Maurer, Ciepla, Chalouni, Franke and Hannoush2016), and showed by NMR its penetration into cells but peptide was retained in the endosome and subsequently degraded. The cell penetration was enhanced by co-incubation of the cell with CPPs which also altered its route in the cell since EETI-II was now found in the cell structures. NMR also revealed the altered intracellular trafficking of the peptide after endocytic internalization. Analogues of penetratin were synthesized using 19F modified amino acids and their penetration was studied by NMR (Christensen et al., Reference Christensen, Kongstad, Sondergaard, Staerk, Nielsen, Franzyk and Wimmer2019). Introducing 19F into the peptide makes possible a more precise following of the fate of the peptide in the cell in comparison to 1H-NMR because of a much better resolution.
Conclusions
The design and improvement of therapeutic CPPs are of great impact in situations where FDA approvals have not been achieved yet. Future development of CPP-based drugs greatly depends on approaches leading to the understanding of CPP mechanisms of (tissue, cellular) uptake. This review summarizes the recent biophysical studies of different aspects on the subject, including the interaction of peptides with interaction partners such as phospholipid membranes and proteins in plasma membrane and circulation as well as in cells.
Characterization of the kinetics of the multistep network of interactions in CPP internalization and CPP-cargo conjugates reveals multiple steps in these complicated interaction networks. Knowing and following the intracellular signaling pathways by biophysical methods demonstrates the endosomal escape of CPP-aided delivery. Promising nucleic acid-based therapies based on CPP-vectorized oligonucleotides (antisense, siRNA, miRNA, and plasmids) and CPP/oligonucleotide nanocomplexes require additional biophysical studies. Intracellular protein–protein or protein–DNA/RNA interactions have been addressed by short protein mimics or proteins using advanced biophysical studies.
The described visualization of CPPs certainly belongs to the biophysical set of CPP research with high impact in the characterization of novel CPP-based therapeutics.
Conflict of interest
None.