1.1 The Wave–Particle Duality and de Broglie Wavelength
It so happens that nature does not always follow the strict rules set by classical mechanics. When attempting to describe phenomena occurring on the nanoscale, this reality becomes most apparent, as we shall see in numerous examples throughout this book. The structure of atoms and molecules, the organization of matter at the atomistic level, the dynamics of charge and energy in different materials, diffraction and scattering of electromagnetic radiation or elementary particles from nanoscale structures, and chemical reactivity are but a few phenomena that cannot be explained within the realm of classical mechanics.
As a concrete example of the breakdown of classical mechanics on the subnanometer length scale, let us consider the scattering of an electron beam from a surface of a metal, as studied by Davisson and Germer in the year 1927 [Reference Davisson and Germer1.1]. The electron beam was initially regarded as a flux of particles (the electrons) in classical terms. Considering the mass and kinetic energy, , each electron is associated with a linear momentum
, where (neglecting relativistic corrections)

In the simplest terms, the metal acts as a perfect mirror when reflecting the electron beam. In the scattering process, the momentum flips its sign in the direction perpendicular to the surface (e.g., ), and consequently, the angle between the direction of the reflected beam and the surface plane (
) equals the impact angle (
), as depicted in Fig. 1.1.1.
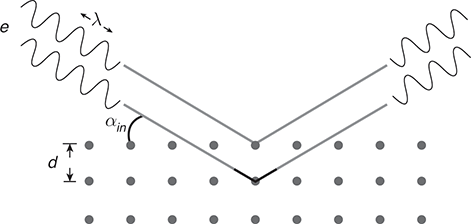
Figure 1.1.1 The classical description of electron beam scattering from a metal surface.
According to this classical interpretation, the reflected flux at should depend on the incoming particle flux, but not on the energy of each particle,
. Yet, in the famous Davisson–Germer experiment a clear dependence of the reflected flux on the energy of the incoming electrons was observed. Moreover, the intensity of the reflected beam showed distinctive maxima at specific E values, which was reminiscent of the result of electromagnetic wave reflection from ordered atomic crystals (X-rays, in particular). This result couldn’t be interpreted within classical mechanics.
In wave theory, modulations in the reflected flux can be readily explained in terms of interference of waves originating from different sources, or incoming through different pathways. When waves are scattered from an atomic crystal, the first and second atomic layers can be regarded as two reflecting mirrors displaced from each other by the interatomic distance, . (Scattering from deeper layers may be neglected for moderate beam energies when the penetration of the beam into the crystal is low.) For particle scattering, the reflection from the two mirrors is additive and therefore equivalent to an effective single mirror. In contrast, for wave scattering, the two mirrors define two scattering pathways with remarkable consequences for the reflected flux owing to interference. In particular, when the two pathways differ in length by an integer multiplicity of the wavelength,
, the interference is constructive, and the reflected beam intensity should obtain a maximum. Given the scattering angle,
, the two pathways differ in length by
(see Fig. 1.1.2). In this case, constructive interference should occur for a set of discrete wavelengths, satisfying the (Bragg) condition:

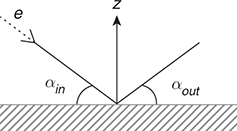
Figure 1.1.2 The wave description of electron beam scattering from a metallic crystal. The pathway of wave scattering from the internal atomic layer is longer by in comparison to wave scattering from the outer layer, resulting in wave interference.
According to the wave theory, the reflected flux should therefore depend on the scattering angle, obtaining a set of maximal values as a function of the wavelength. But how could such a theory apply to a beam of particles? How could one relate the modulation in the particle’s energy to modulation in some respective wavelength? The experimentally observed similarities between scattering of electrons and of electromagnetic waves by a metal crystal led Davisson and Germer to conclude that [Reference Davisson and Germer1.1] “a description of the occurrence and behavior of the electron diffraction beams in terms of the scattering of an equivalent wave radiation by the atoms of the crystal, and its subsequent interference, is not only possible, but most simple and natural.” Indeed, it was already proposed by Louis de Brogliede-Broglie in 1924 [Reference de Broglie1.2] that a beam of particles (electrons, included) can be associated with a wavelength, according to the following postulate:

where is Planck’s constant (already known by then, from the theory of electromagnetic radiation [Reference Planck1.3]), and
is the particle’s momentum in the direction of the beam propagation. The Davisson–Germer experiment reinforced a dual description of an electron beam in terms of both particles and waves. The maxima in the reflected beam flux as a function of the electron energy (
) could be correlated with wave properties not only qualitatively, but also quantitatively. By implementing the conditions for constructive wave interference (such as Eq. (1.1.2)), the de Broglie relation between momentum and wavelength (Eq. (1.1.3)), and the classical relation between the momentum and energy (Eq. (1.1.1)), that is,
, the maxima in the reflected flux as a function of the kinetic energy of the electrons could be explained.
Notice that while the wave properties of the electron beam turn out to be inherent to its nature, their manifestation in the experiment required special conditions. For the different interference peaks in the reflected flux to be distinctively resolved, the ratio should not be too small. From Eq. (1.1.2) it follows that this ratio equals
, which means that the wave properties are most clearly pronounced for
, or in physical terms, when the de Broglie wavelength of the electrons is of the order of the interatomic distance,
. As the de Broglie wavelength of the electrons becomes smaller (
), for example, at higher kinetic energies,
becomes too large to enable resolving specific interference peaks. Therefore, the discovery of the wave nature of the electron required that the de Broglie wavelength (set by the kinetic energy) would be of the order of the (subnanometer) interatomic distances in the atomic crystal. Moreover, the quantitative verification of de Broglie’s relation (Eq. (1.1.3)) was based on prior knowledge of the interatomic distance (
), which was already inferred from X-ray diffraction measurements. Remarkably, it took more than two centuries after the establishment of classical mechanics for the revelation of the electron’s wave–particle duality. The characterization of the structure of matter on the subnanometer scale was an essential precondition for this discovery.
The distance between adjacent atomic layers in a nickel crystal is meter. An electron beam is scattered from the face of the crystal at an angle,
. Given the electron mass,
kg, calculate the kinetic energy for the three most resolved maxima in the reflected flux at
(see Figs. 1.1.1 and 1.1.2).
In the “classical world,” the de Broglie wavelength is typically much smaller than the characteristic length scale of the system under consideration. Consider, for example, a tennis ball at a mass kg, flying at a typical serve-velocity, for example, 50 meter/sec. What is the associated de Broglie wavelength? How does it compare with the length of a tennis court (24 meter), or with the diameter of the ball itself (6.7 cm)?
1.2 Quantum Mechanics for Nanoscience and Engineering
Quantum mechanics, as detailed in the following chapters of this book, provides a theoretical framework that accounts consistently and comprehensively for the wave–particle duality. Indeed, any matter is associated with wave properties, which are expressed most profoundly when the associated de Broglie wavelength is of the order of the characteristic length scale of the system under consideration,

In the realm of nanoscience and engineering, the characteristic length scales are associated with the typical dimensions of atoms ( m), molecules (
m), or nanocrystals (
m). The relevant masses are those of electrons (
kg) and small atoms (
kg), and the relevant kinetic energy scales are typically
eV. Translating the masses and energy values to wavelength according to de Broglie (Eq. (1.1.3)), it immediately follows that the condition for manifestation of wave properties, Eq. (1.2.1), is often fulfilled. Quantum mechanics is therefore essential for a proper description of phenomena associated with the nanoscale. These include, for example, the colors of matter (absorption spectra of atoms, molecules, nanoparticles, etc.), electrical conductivity, interatomic forces, the structure of matter, chemical reactivity, heat and energy transport, and many more.