Book contents
- Fetal and Neonatal Brain Injury
- Fetal and Neonatal Brain Injury
- Copyright page
- Contents
- Contributors
- Preface
- Section 1 Epidemiology, Pathophysiology, and Pathogenesis of Fetal and Neonatal Brain Injury
- Section 2 Pregnancy, Labor, and Delivery Complications Causing Brain Injury
- Section 3 Diagnosis of the Infant with Brain Injury
- Chapter 16 Clinical Manifestations of Hypoxic-Ischemic Encephalopathy
- Chapter 17 The Use of EEG and aEEG in Assessing the Term and Preterm Brain
- Chapter 18 Neuroimaging in the Evaluation of Pattern and Timing of Fetal and Neonatal Brain Abnormalities
- Chapter 19 Light-Based Assessment of the Brain
- Chapter 20 Placental Pathology and the Etiology of Fetal and Neonatal Brain Injury
- Chapter 21 Timing Perinatal Hypoxic-Ischemic Brain Injury
- Section 4 Specific Conditions Associated with Fetal and Neonatal Brain Injury
- Index
- References
Chapter 21 - Timing Perinatal Hypoxic-Ischemic Brain Injury
from Section 3 - Diagnosis of the Infant with Brain Injury
Published online by Cambridge University Press: 13 December 2017
- Fetal and Neonatal Brain Injury
- Fetal and Neonatal Brain Injury
- Copyright page
- Contents
- Contributors
- Preface
- Section 1 Epidemiology, Pathophysiology, and Pathogenesis of Fetal and Neonatal Brain Injury
- Section 2 Pregnancy, Labor, and Delivery Complications Causing Brain Injury
- Section 3 Diagnosis of the Infant with Brain Injury
- Chapter 16 Clinical Manifestations of Hypoxic-Ischemic Encephalopathy
- Chapter 17 The Use of EEG and aEEG in Assessing the Term and Preterm Brain
- Chapter 18 Neuroimaging in the Evaluation of Pattern and Timing of Fetal and Neonatal Brain Abnormalities
- Chapter 19 Light-Based Assessment of the Brain
- Chapter 20 Placental Pathology and the Etiology of Fetal and Neonatal Brain Injury
- Chapter 21 Timing Perinatal Hypoxic-Ischemic Brain Injury
- Section 4 Specific Conditions Associated with Fetal and Neonatal Brain Injury
- Index
- References
Summary
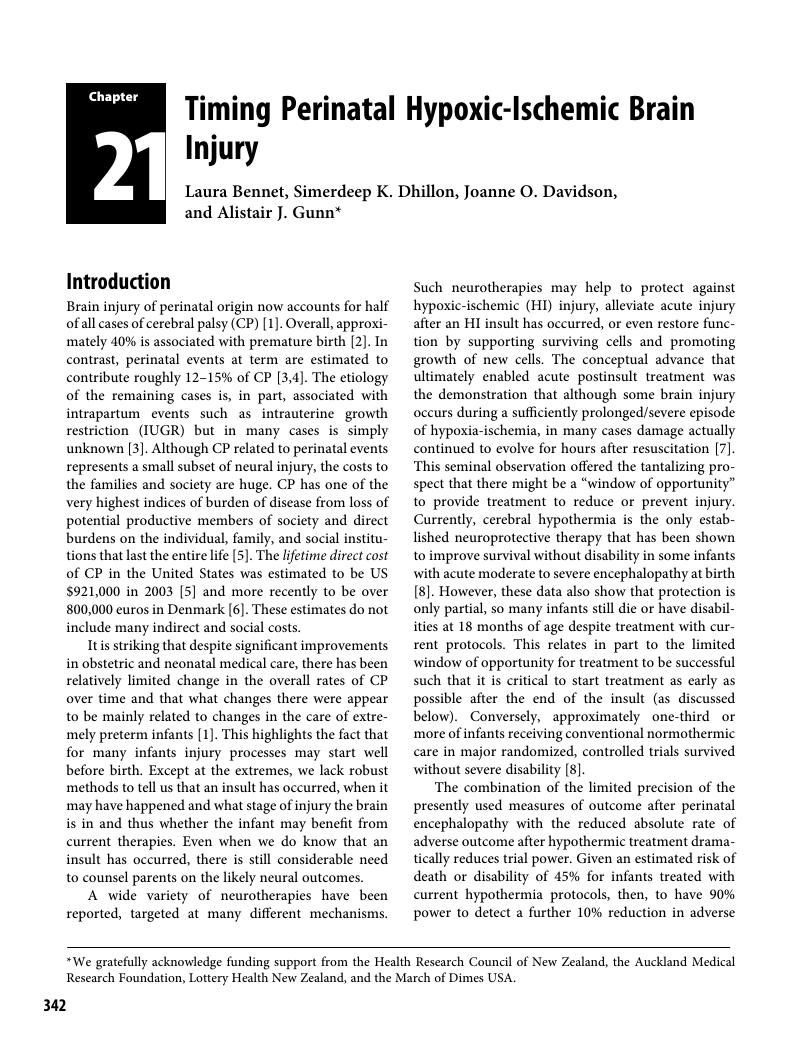
- Type
- Chapter
- Information
- Fetal and Neonatal Brain Injury , pp. 342 - 356Publisher: Cambridge University PressPrint publication year: 2017